Fig. 14.1
A taxonomy of hand functions spanning a spectrum from precision to stability
Historically, the ability to control each digit individually while keeping other digits immobile has been used as a measure of the flexibility needed for fine digit control. One attempt to quantify individuation ability was introduced by Schieber [31], who emphasized the importance of being able to keep nonmoving digits stationary while moving one digit independently. This approach, however, does not take into account the control of force. An alternative approach is to measure individuation ability in an isometric force generation task [32, 33]. Individuation ability in such tasks is modulated by the level of output force: the exertion of greater force compromises individuation.
The loss of individuation ability in terms of increased enslaving with higher level of force production in the active finger is often described as the transition from a precision to a power grip. We will discuss this “neuromuscular coupling” effect [34–37] later in the chapter. We will show that although pure individuation of the digits is hardly found in natural hand actions, it can be especially useful for evaluating impairment and recovery of hand function after stroke [38, 39].
Between the two extremes of pure individuation and power grip, precision pinch has also been extensively studied. For example, Valero-Cuevas and colleagues have used a strength-dexterity paradigm to examine the dynamics of both force and motion control at the finger tip (e.g., [19, 40–42]). In their task, participants are asked to use their finger and thumb to bring two flat discs together by compressing a spring that connects them. By parametrically varying the size and stiffness of the spring, the interplay between movement and force control can be investigated systematically. An important conclusion that emerges from this series of studies is that the control principles for moving the finger and for applying static force are qualitatively distinct and the task requires the need to quickly switch between these two modes of control. The two types of controller require different muscle coordination strategies [43, 44]. Thus fine digit control involves the control of both force and movement in all fingers as well as the dynamic transition between the two to achieve stability and precision. Understanding these phenomena in healthy adults may provide insight into the mechanisms of impairment and recovery following stroke.
14.2.2 Hierarchical Control of the Hand
Motor control of the hand is achieved in a hierarchical manner: commands are sent from the central nervous system (CNS) to the muscles; the muscles pull on tendons and bones to generate different joint torques; these then generate movement and contact forces that allow it to move through and interact with the environment to achieve specific task goals (Fig. 14.2). These many layers confer considerable flexibility on the motor system. Usually, we can achieve any given motor task in a number of different ways. This property is known as redundancy. The redundancy problem (or the degrees of freedom problem) in motor control [45] was originally introduced to refer to the question of how our brain chooses a particular limb configuration and movement among the seemingly infinite possible solutions to achieve a specific task goal.
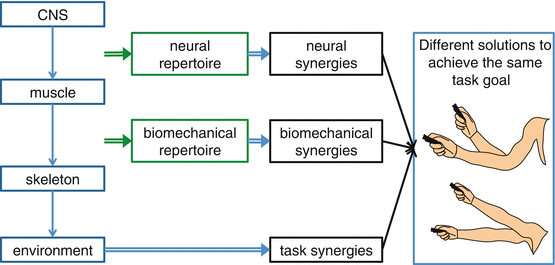
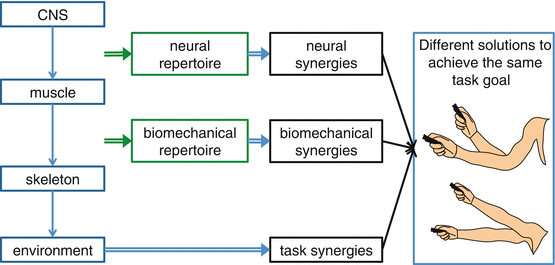
Fig. 14.2
Hierarchical control
Redundancy can exist at all points of the control hierarchy. Suppose you intend to push a button. This requires a (relatively) unique endpoint force to be applied to the button. However, you could choose any one of ten fingers to push the button with. For any given finger choice, multiple postures of the hand and arm could be used. We typically have more muscles than degrees of freedom, so that the same endpoint force can often be achieved using many different muscles [46, 47]. Finally, the same muscle activations can be achieved by multiple distinct patterns of activity in the CNS [48].
Interestingly, it has been shown that there is less muscle redundancy for fine digit control than was previously thought. Only about 6–23 % of the 2D force space for a fingertip is robust to the loss of some muscles; and this space becomes even smaller at higher force levels [49]. The extent of redundancy at the musculoskeletal level is the key mechanical difference between the arm and the hand. For example, multiple patterns of shoulder and elbow muscle activation can produce similar torque and joint angle kinematics during reaching [46, 47]. The implication of these arm and hand differences in biomechanical redundancy for neural control is unclear, but it is of interest that reaching has been achieved by many mammals whereas individuation of digits has not. We shall see later in the chapter (Sect. 14.4.2) that motor cortex in primates developed a monosynaptic connection that is correlated with digit individuation.
14.2.3 Synergies and Repertoire
The presence of redundancy in the motor system has historically been considered a problem. The problem of which action to select to achieve a given task may seem straightforward at the level of the effector, but could become overwhelming at the level of the CNS. It has often been suggested that the brain may use simplifying control strategies to aid in solving this problem. This led to the notion of motor synergies—simplified building blocks from which the brain can construct movements. The idea of synergy is used extensively in both the motor control and clinical stroke literature. However, the term is inconsistently applied across the two fields, causing considerable confusion. Some clarification comes from borrowing two definitions of synergy provided by Diedrichsen et al. [50]. First, synergies can be identified as an empirical fact, in which case synergy refers to systematic co-articulation of different joints or coactivation of different muscles, observed over a set of behaviors. This empirical definition of synergies is quite distinct from the notion of synergy as an explanatory concept, a hypothetical control structure or module in the motor system that activates muscles or effectors as a single unit.
A common observation following stroke is a loss of ability to move individual joints in isolation. Patients exhibit apparently obligatory patterns of multi-joint motion. These co-articulations are often described as synergies. For the arm, these are the classical flexor and extensor synergies [51]; for the hand, power grip is often retained at the expense of digit individuation. While these synergies clearly conform to an empirical definition, it is unclear what the functional significance of these synergies is.
Although synergies in the explanatory sense have often been thought of reflecting building blocks through which to synthesize movements, another way to think of synergies in this sense is as a constraint on potential actions (Fig. 14.2). Consider the enslavement of a finger when attempting to individuate a neighboring finger. While this co-actuation could be described in terms of a synergy, it could equally be thought of as a constraint on the commands that can be issued by the CNS, limiting the repertoire of available actions. We believe that, in the context of stroke, the notion of repertoire offers a more illuminating way to frame co-articulation. During normal function, repertoire places an emphasis on what can and cannot be achieved by a particular level in the control hierarchy acting on a lower level, rather than on the nature of individual building blocks. Following stroke, it makes more sense to think in terms of losing repertoire than gaining synergies. In the extreme, it would be odd to describe paresis as a synergy, but it is naturally characterized as a catastrophic loss of repertoire.
A further difficulty in the use of the term “synergy” is the considerable confusion that can arise with regard to the source of the synergy. Although an empirically observed synergy could potentially reflect a limited repertoire with which the CNS can actuate the hand (neural synergy), in many cases, there can be other ways to explain such co-articulation or co-actuation. To illustrate these distinctions consider the simple task of using a laser pointer to draw a vertical line on the wall (Fig. 14.2). There is an infinite set of solutions in terms of how to distribute rotation around the wrist, elbow, and shoulder joints. Biomechanical constraints may render it impossible to achieve certain kinematic postures or movements and may be sufficient to explain the phenomenon of synergies (biomechanical synergy) [52]. For instance, if the range of motion around particular joints was limited or muscle insertions were such that one joint could not be moved in isolation, this would restrict the biomechanical repertoire, and particular synergies would arise for this reason alone.
However, apparent synergies could also derive from higher-order movement strategies or from task constraints (task synergy). In the laser pointer example, at the task level, some possible constraints could be the need to draw a vertical line of a given length and the details on how the line is to be executed, for example, fast, accurately, or multiple times. These constraints may directly lead to apparent synergies in a similar way to direct biomechanical constraints. Even in an entirely unconstrained setting, it may be more energetically efficient or it may minimize the impact of execution if one distributes movement of the hand across all joints evenly. In this case, there would be an apparent synergy, although it would reflect a specific control strategy rather than a constraint on repertoire. Synergies may therefore emerge from an optimal solution to a given task without necessarily reflecting biomechanical or neural constraints [50, 53].
14.3 Neural Control of the Hand
14.3.1 Cortical Representation
One place to look for an explanation for the remarkable dexterity of the human hand is in motor cortex. A longstanding question with regard to cortical hand control is whether there are distinct representations for individual digits and, if so, how these representations are organized. A study using transcranial magnetic stimulation (TMS) showed that the cortical sites that can evoke individual finger movements appear to be distributed and overlap extensively [54]. Diedrichsen et al. [33] (as well as [55–59]) showed distributed and overlapping digit representation in motor cortex using fMRI. Corroborating results have been obtained in nonhuman primates [60–62]. For example, Schieber and Hibbard [63] found that cortical neurons governing finger movements are distributed throughout the hand-control area of the primary motor cortex rather than being functionally segregated.
In addition to single-digit representations, there is also evidence to suggest that more complex actions are represented in motor cortex. TMS can evoke stereotypical hand postures phenotypically similar to voluntary grasping movements [54]. In a follow-up study, the same group showed that TMS evoked hand synergies (in the empirical sense) relevant to playing of the violin in well-practiced violinists but not in nonmusicians, which suggests that unique representations can be constructed in motor cortex. Using microstimulation, Overduin et al. [64] were able to elicit stereotypical muscle activation patterns from macaque monkeys’ rostral motor and caudal premotor cortices. As in the noninvasive human studies, these patterns of muscle activation were similar to those found in the monkeys’ natural behaviors [65].
Thus it appears that motor cortex is equally capable of controlling single digits and constructing multi-digit synergies. In other words, it seems to possess a broad repertoire for hand control. The distributed cortical organization suggests that it is artificial to draw a distinction between moving a single digit and moving several digits in a particular way, a distinction that stems from the misleading intuition that each finger should have a separate island of representation. For any pattern of finger movement, control of all the digits is required. Even in the single-digit case, movements of other digits need to be suppressed, which can only be accomplished through multiple muscle activations [66]. It is therefore clear that the term individuation is not synonymous with moving a single digit but instead refers to the contrast between power grip and complex patterns of multi-digit movement; single-digit movement is just a special case of the latter.
14.3.2 Descending Pathways for Hand Control
The combinatorial complexity of representation in motor cortex would be of little benefit unless the bandwidth exists to transmit the information to the muscles of the hand. Evolutionarily, expansion in primary motor and sensory cortices has been accompanied by the emergence of a monosynaptic cortico-motoneuronal (CM) connection. Using retrograde transneuronal transport of rabies virus, Rathelot and Strick [62, 67] found that cortical neurons possessing monosynaptic connections to the spinal cord are clustered in caudal regions of primary motor cortex, in the anterior bank of the central sulcus, and directly project to the ventral horn of the spinal cord (Fig. 14.3). This has been called “new” M1, because of its recent emergence in evolutionary terms. There is a great deal of convergent evidence supporting the idea that these monosynaptic spinal projections are critical for finger control [14, 15, 38, 68–74]. Most famously, in a classic study, loss of precision pinch occurred after bilateral interruption of medial and lateral pyramidal tracts in rhesus monkeys, but walking, running, climbing, and gripping abilities, as well as strength were largely preserved [75, 76]. In contrast, after ventromedial brainstem lesions, disrupting reticulospinal and vestibulospinal descending pathways (projecting down from “old” M1 circuitry), monkeys showed striking impairment in walking and climbing, and limited movement of the head, trunk, and shoulder, but relatively preserved hand function.
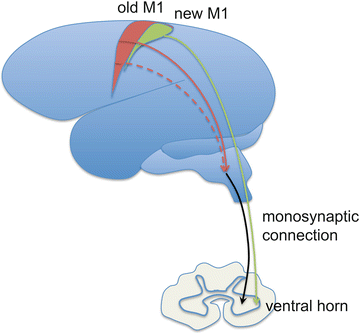
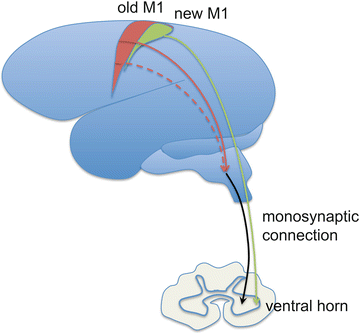
Fig. 14.3
Illustration of the old vs. new M1 and their differential projections to motoneurons in the spinal cord
These findings powerfully illustrate how the overall repertoire of the motor system is divided across different brain regions. It appears that reticulospinal pathways descending from the brainstem possess a repertoire tuned for gross, proximal movements, but a very limited repertoire for hand control. Thus it appears that “old” M1 circuitry, at least in the nonhuman primate, may modulate brainstem and spinal controllers for reaching and power grip. Corticospinal pathways containing the monosynaptic projections from “new” M1 neurons to the spinal cord can bypass this limited brainstem repertoire. “New” M1 may therefore possess a far broader repertoire for hand movements and may be the critical component for control of the hand and for more human-specific proximal behaviors like throwing a Frisbee.
The exact mechanism by which the monosynaptic connection confers dexterity is still unclear. A clue comes from the observation that monosynaptic connections have their lowest threshold and largest amplitude activation effect on the intrinsic muscles of the hand (comparing to more proximal muscles) [14, 77]. A second role is suggested by findings of Valero-Cuevas and colleagues [78, 79] demonstrating that the transition between movement and isometric phases of precision pinch requires anticipatory time-critical switching between two kinds of neural controllers, precise timing that could best be provided by a short-latency connection. Lemon [77] also pointed out that flexibility is one of the most fascinating features of the CM-muscle connections and suggested that combined with the overlapping cortical output to the CM neurons, these features likely subserve dynamics and stability for movement of the whole limb.
14.4 The Nature of the Initial Impairment in the Hand After Stroke and Its Subsequent Recovery
14.4.1 Dissociation of the Impairment of Strength and Control
Stroke causes characteristic abnormalities in the hand that reflect the neural organization of hand control. Weakness is very common after stroke especially in the finger extensors [39, 80–82], and wrist muscles [83], and seems to be the primary contributor to impairment in moderate to severe stroke [84]. For any level of weakness there is also loss of control. In severe paresis, weakness and loss of control amount to the same thing, but in mild to moderate hand paresis, they can dissociate both at onset and as recovery ensues. The weakness is due to loss of input into motoneurons, whereas loss of control can be attributed to the combination of weakness, spasticity, and altered patterns of muscle activation. For example, failure to extend the fingers is thought to result from both weakness and unwanted coactivation of finger flexor and extensor muscles, likely due to motoneuron hyperexcitability and loss of reciprocal inhibition [82, 84–86]. Altered patterns of muscle activation are evident in the relative preservation of finger flexion during grasp compared to finger and wrist extension and individuation after stroke [38, 39]. The impairment of finger individuation results both from reduced ability to isolate the appropriate muscle [86, 87] and increased unwanted force production in task-irrelevant fingers [37].
Dissociation between grip force and control of individual fingers is strikingly illustrated by the fact that the ipsilesional hand can show abnormalities in dexterity despite preserved grip strength after stroke [88], consistent with recent demonstration of the role of both hemispheres for finger control [33, 89–93]. The dissociation between weakness and control seen in the hand after stroke is also recapitulated in the time-course and degree of recovery. Heller et al. [94] showed that grip strength recovered earlier than other aspects of hand function such as the ability to manipulate objects. Similarly, Sunderland et al. [95] showed better return of grip strength compared to dexterity as measured by the Nine Hole Peg Test. It should be emphasized that of course some degree of strength is necessary for control [96] and so it would be incorrect to assume that they recover entirely independently. That said, it is a common observation to see a patient able to perform a power grip with all the fingers but unable to flex a single finger while keeping the others still.
14.4.2 Neural Basis for Hand Impairment After Stroke
A major challenge for stroke research is to explain the neural basis for both the initial hand abnormality after stroke and the manner in which it recovers. We shall focus here on changes in control from cortical and brainstem areas and the balance between them. Total loss of strength in the hand (plegia) can be observed after an isolated stroke in M1 or in white matter projections such as the posterior limb of the internal capsule. The striking similarity of the abnormality to classical pyramidal lesions in monkeys [75] strongly implicates a corticospinal contribution to the phenotype. As described above, however, relative preservation of finger flexion, especially power grip can be observed initially after mild to moderate stroke and power grip strength returns before finger dexterity during recovery. Two types of explanation, which are not mutually exclusive, have been put forward for those residual features of the post-stroke hand phenotype. One posits recruitment and facilitation of residual corticospinal neurons, and the other posits cortical modulation of brainstem descending pathways.
A clue as to the neural basis of hand impairment post-stroke comes from the observation that, even in healthy subjects, there is reduction in individuation when increased force is required [35, 97–102]. That is to say, at high force, the force vs. dexterity trade-off seen in hand paresis can be recapitulated in healthy subjects. One classical finding that sheds light on this phenomenon is that some CM cells in M1 are activated when the muscle they are innervating is involved in precision grip but not when the same muscle is involved during a power grip [103]. Evidence suggests that the CM connection is not responsible for high levels of force production, but rather for small finely graded forces [104]. In humans, using multivariate fMRI method, Diedrichsen et al. [32] also confirmed that while BOLD activation scales with force levels, higher force production also recruits larger cortical areas in M1. A possible explanation for this finding is that brainstem descending pathways are differentially recruited at high force levels. Indeed, there is evidence that the reticulospinal system may become more important for strong contractions [105]. Axons of the reticulospinal tract branch more extensively within the spinal cord than corticospinal axons, contacting more motoneuron pools, and consequently sacrificing repertoire for force [106, 107]. Thus a potential mechanism for loss of individuation after stroke but preservation of power grip is through early recruitment of reticulospinal pathways to maintain force levels. This conjecture is consistent with increased mirror movements in patients after stroke (the reticulospinal tract projects bilaterally) and from the demonstration of a similar loss of individual control of elbow and shoulder joints following recruitment of reticulospinal connections in patients after stroke when the limb must generate force to counter gravity, but not otherwise [108].
Thus a dynamic interplay between preserved monosynaptic corticospinal projections and relatively intact reticulospinal projections appears to be the basis both for the initial paresis phenotype and subsequent recovery patterns. Initial plegia after stroke is likely due to loss of corticospinal input from M1 and a delay before other descending pathways and reticulospinal projections are facilitated. Recovery from cortical infarcts, especially small ones, is better than after subcortical strokes with comparable initial degrees of paresis. This is thought to be because of redundancy in representation at the cortical level [109, 110]. In a recent paper, Darling and colleagues [111] investigated effects of fairly extensive motor cortical lesions in macaques. They observed quite remarkable return of dexterity, measured by having the animal pick small food pellet from wells of different size, occurring up to 3 months after the induced stroke. One outstanding question is whether there was some sparing of new M1 deep in the central sulcus in some of these monkeys, which might explain the impressive return of dexterity despite the extensive nature of the motor cortical stroke.
We conjecture that the impressive degree of recovery in the nonhuman primate models compared to human stroke is the concomitant involvement of white matter infarction in the latter. Isolated lesions of the posterior limb of the internal capsule, in essence the inverse lesion to those studied by Darling and colleagues [111], constitute a concentrated white matter lesion with no cortical component. These lesions can cause lasting dense paresis, presumably because all motor corticofugal pathways are interrupted. Capsular lesions in humans cause a phenotype that is very similar to what is seen in nonhuman primate pyramidal lesions [88]. In a very interesting recent study, extensive unilateral lesions of the medullary pyramid were made in three adult macaque monkeys [112]. After an initial contralateral flaccid paralysis, power grip returned but individuation did not. Stimulation of a site in the brainstem (specifically, the medial longitudinal fasciculus in the medulla, a site from which many descending reticulospinal axons can be activated) revealed facilitation of mono- and disynaptic excitatory postsynaptic potentials after recovery, but only in motoneurons innervating forearm flexor and intrinsic hand muscles, not in forearm extensor motoneurons. This finding showed, for the first time, the existence of reticulospinal tract facilitation of the hand and clearly demonstrates the limitations inherent in these pathways for subserving hand function. It is also striking how the pattern of return of both excitatory potentials and selective aspects of hand function mimic what is seen in patients with severe hemiparesis: preservation of flexion for grip but persistent weakness in extensors.
Thus, on balance, it seems that recovery of hand dexterity is very much dependent on the degree to which there is a sparing of contralateral descending pathways. If these are extensively damaged, then only a limited degree of recovery is possible within the capacity of reticulospinal connections, presumably under modulatory control from “old” M1 and other cortical areas. What we do not know is whether the balance between corticospinal and reticulospinal recovery is subject to manipulation in those cases of subtotal lesions where both pathways could be facilitated. Data certainly suggest that the potential for significant recovery from cortical lesions is dependent on training [111, 113].
14.4.3 True Recovery vs. Compensation
The distinction between true recovery and compensation is important for both thinking about and guiding neurorehabilitation. Compensation is task specific, goal-directed, and achieved by exploring alternative movement patterns; recovery is general and involves regaining, at least partially, the original movement patterns used to accomplish a given task [114–118]. Referring back to the hierarchical control of the hand, compensation can be considered achievement of success at the task despite a loss of neural repertoire. Here we operationally define true recovery as regaining the full repertoire of movements that was available before injury. We use compensation to refer to improved performance in a task by finding better ways to achieve it given a diminished repertoire, for instance, using the unaffected hand to perform a task that would have been performed by the affected hand prior to injury. However, more subtle versions of this distinction can exist within effectors or, in general, wherever a task can be achieved in multiple ways.
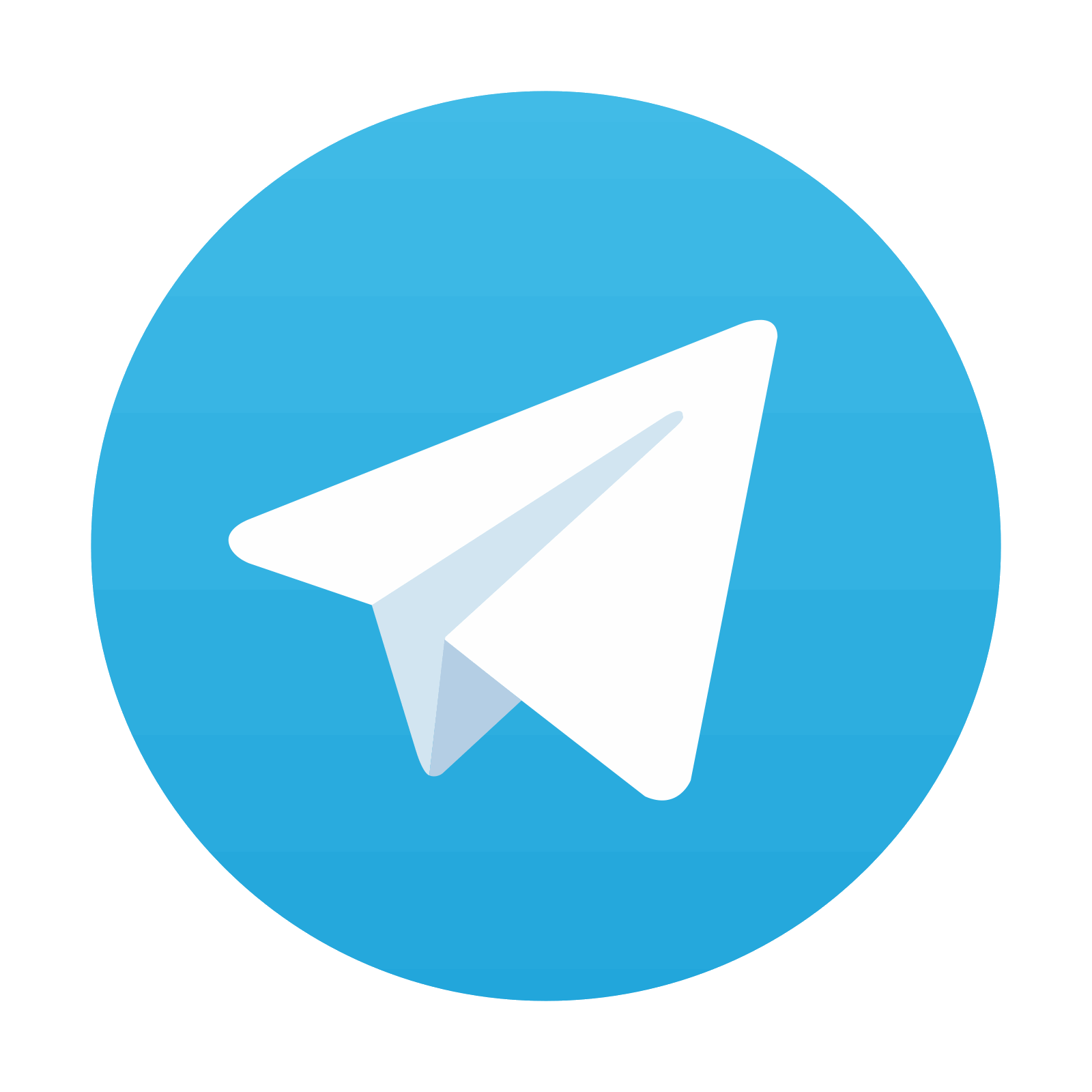
Stay updated, free articles. Join our Telegram channel
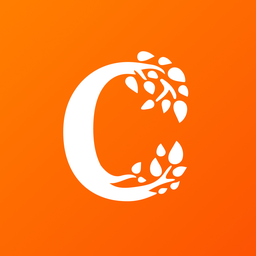
Full access? Get Clinical Tree
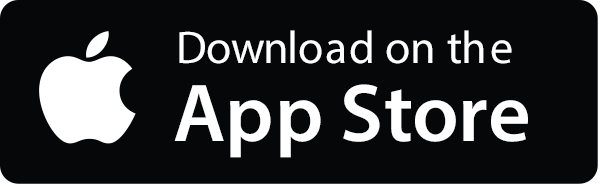
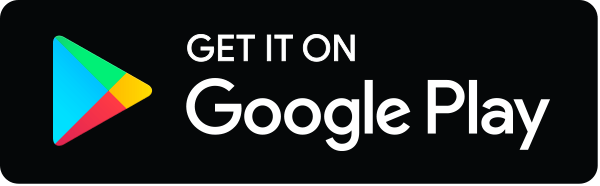