41111 Multimodal Monitoring and the Ictal-Interictal Continuum LEARNING OBJECTIVES • To describe multimodality neurophysiologic monitoring • To become familiar with noninvasive and invasive techniques for neurophysiologic monitoring in the pediatric neurocritical care unit • To describe EEG patterns on the ictal-interictal continuum Introduction The goal of pediatric neurocritical care (NCC) is to improve the outcome of patients with life-threatening neurologic illness. The diagnosis and management of primary brain injury, for example, anoxia, intracranial hemorrhage, ischemic infarction, meningoencephalitis, and traumatic brain injury, among others, is of critical importance in the intensive care unit. However, the prevention, early detection, and mitigation of secondary brain injury due to cerebral edema, cerebral ischemia, and/or increased intracranial pressure, among others, is just as important as the diagnosis and management of the primary injury. Multimodal neurologic monitoring plays a key role in enabling the prevention, detection, and mitigation of secondary injury in critically ill patients. While the neurologic examination is a key component of comprehensive neurologic monitoring, this is often limited in acute brain injury by coma, sedation, and pharmacologic neuromuscular blockade. Moreover, by the time the neurologic examination changes, secondary injury may have become irreversible. Real-time neurophysiologic monitoring is necessary to detect brain injury early, at a stage when acute intervention may prevent irreversible injury. When applied to the prevention of secondary brain injury, multimodal neurologic monitoring, including continuous EEG (cEEG) monitoring, has the ability to “detect and protect.”1 This chapter reviews the role of invasive and noninvasive techniques, including cEEG, that allow for neurologic monitoring in critically ill children, with the ultimate goal of detecting and treating injury early and thereby potentially improving patient outcomes. Multimodality Monitoring Multimodality monitoring (MMM) refers to the assessment of multiple physiological parameters using combinations of noninvasive and invasive techniques to augment the neurologic examination.2–7 The purpose of MMM, similar to that of intraoperative monitoring, is to detect early signs of neurologic deterioration and intervene, if possible, in a timely manner to prevent irreversible neuronal injury. MMM can be used to individualize patient management, assess response to treatment, and modify subsequent treatment to prevent further injury.6 A recent consensus on MMM identified physiologic data and processes important to neurocritical care (NCC) these include: the clinical examination; systemic hemodynamics; intracranial pressure and cerebral perfusion pressure; cerebrovascular autoregulation; systemic and brain oxygenation; cerebral blood flow and ischemia; electrophysiology; cerebral metabolism; glucose and nutrition; hemostasis and hemoglobin; temperature and inflammation; and biomarkers of cellular damage and degeneration.2 MMM uses both invasive and noninvasive monitoring techniques. Noninvasive monitoring techniques include: cEEG, near-infrared spectroscopy (NIRS), and transcranial Doppler (TCD) ultrasonography. Invasive monitoring techniques include intraventricular and parenchymal devices to directly measure intracranial pressure (ICP), cerebral blood flow (CBF), and brain tissue oxygenation (PbtO2), microdialysis catheters to measure CSF substrates (i.e., lactate, pyruvate, etc.), and depth electrodes (DE) to allow for invasive electrophysiologic monitoring. While most MMM done to date has been in adults, MMM has been introduced in pediatric NCC.8 Several important reviews are used to inform the discussion of these techniques.2–7 Specific references are indicated for additional information. Electroencephalography Continuous EEG cEEG monitoring in the ICU expanded markedly with the introduction of digital technology for EEG. With analogue recording, a “routine” EEG done intermittently was used to identify seizures, assess background, or monitor the degree of burst-suppression during treatment with pentobarbital for status epilepticus or increased intracranial pressure. Continuous EEG monitoring with video represents a significant advance over serial analog EEGs. It allows for better identification of subtle clinical seizures and subclinical seizures, and it allows for quantification of seizure burden. It also enables the detection of acute changes in background patterns, given that it is performed 412continuously, rather than intermittently. Moreover, while the waveforms that constitute the ictal-interictal continuum were identified on “routine EEG,” cEEG identified their dynamic nature and permitted a much more detailed and nuanced understanding of this continuum. Quantitative EEG The addition of quantitative EEG (qEEG) and digital trending analysis to cEEG monitoring has greatly enhanced the ability to identify EEG changes over time. Digital trending is derived from and time-locked to the cEEG. MMM is usually performed concomitantly with cEEG and digital trending, and certain software packages allow for simultaneous visualization of the cEEG, digital trends, and other physiologic parameters. cEEG and qEEG are well covered elsewhere in this text and will be discussed in this chapter only as they relate to MMM and the ictal-interictal continuum. Noninvasive Monitoring Techniques Systemic Hemodynamics Heart rate, blood pressure, mean arterial pressure, and pulse oximetry are used for monitoring cardio-respiratory function, in addition to informing the assessment of intracranial hemodynamics.9,10 Temperature It is important to prevent hyperthermia as hyperthermia is associated with worsened outcomes in patients with acute brain injury.11 NIRS Near-infrared spectroscopy uses infrared light to estimate brain tissue oxygenation using the relative absorption of oxygenated and deoxygenated hemoglobin (Hb). The ratio of oxygenated Hb to total Hb, the tissue oxygenation index, is related to regional CBF. NIRS has a long history of use in the neonatal and cardiac intensive care units and during cardiac surgery. NIRS is an indirect measurement of CBF. TCD Transcranial Doppler ultrasonography indirectly measures the mean blood flow velocity of red blood cells (RBCs) in major intracranial arteries.12–14 TCDs have been used in the assessment of children with TBI; intracranial hypertension; vasospasm, stroke, and other cerebrovascular disorders; CNS infections;15 extracorporeal membrane oxygenation (ECMO);16 and brain death. TCDs can be used as a tool to identify patients that may benefit from invasive ICP monitoring or digital subtraction angiography. Pupillometer The pupillometer is a hand-held device that can provide an objective measure of pupil size and reactivity before and after application of a light stimulus. In the management of patients with suspected disorders of ICP, abnormalities of pupil reactivity are often associated with neurologic deterioration and poor outcome.17 Abnormal pupil reactivity is associated with cerebral herniation, oculomotor nerve compression, and brainstem hypoperfusion.18 Traditional means of monitoring pupil size and reactivity by gross inspection are highly subjective,19 leading to the need for more objective means of detecting changes. Aside from providing objective measurements of pupil size, the pupillometer can calculate pupil reactivity (NPi), latency time, and constriction and latency velocities.20 Characterization of changes within these variables may be helpful in identifying pathological rises in ICP in select patients, and attempts have been made to establish pediatric normative data.19 Invasive Monitoring Techniques Invasive multimodality monitoring includes intraventricular and parenchymal devices to measure intracranial pressure, cerebral blood flow, and brain tissue oxygenation (PbtO2), cerebral microdialysis to evaluate cerebral biochemistry (e.g., CSF lactate, glucose), and depth electrodes for invasive EEG monitoring. These yield parameters such as ICP, CPP, brain tissue oxygenation, pressure reactivity, and chemical concentrations of the dialysate. Intracranial Pressure Intracranial pressure is the pressure generated by the major contents of the intracranial space: brain tissue, cerebrospinal fluid, and arterial and venous blood. The skull is a fixed space, so when the volume of one component increases, there must be a compensatory decrease in the volume of the other components, or the ICP will increase. Certain types of intracranial pathology, for example, space-occupying lesions, increase the volume of the intracranial contents and may result in an increase in ICP if compensatory mechanisms are overwhelmed. ICP is typically measured from the brain parenchyma or intraventricular space through an intraparenchymal probe or external ventricular drain, 413respectively. The ICP waveform is synchronized to each beat of the cardiac cycle and can be analyzed for normal and abnormal components. Normal waveform components include: P1, the percussion wave, which represents arterial input at peak systole; P2, the tidal wave, which represents the venous pulsation just prior to the dichrotic notch (e.g., closure of the aortic valve); and P3, the dichrotic wave, which represents venous drainage immediately after the dichrotic notch. The origin of the ICP waveform is primarily arterial, with retrograde venous pulsations contributing to the latter components. As intracranial pressure increases and brain compliance decreases, the pulse amplitude of the ICP waveform increases and P2 increases above P1 (Figure 11.1). Paroxysmal increases in ICP with a broader time scale are called Lundberg waves. The A wave, called the Lundberg wave or the plateau wave, represents an increase in ICP to ~50 mmHg for 5–20 min followed by a spontaneous decrease in ICP. The A wave occurs when autoregulation is intact but brain compliance is decreased. B waves are transient sharply peaked waves to pressure levels of 30 mmHg, which frequently recur and are related to the respiratory pattern; B waves also signify abnormal compliance. C waves, rhythmic elevations occurring every 10 min, are associated with fluctuations in intracranial arterial blood volume and are not considered pathologic (Figure 11.2). In adults, the threshold for treating elevated ICP is 20–25 mmHg.21 In children, the Brain Trauma Foundation “supports the use of less than 20 mmHg as the initial ICP target” and “supports the need for intervention when ICP is raised greater than 20 mmHg for 5 minutes.” Increases for less than 5 min may not be significant; however, increases for >5 min may be significant and treatment should be considered.21 CPP The cerebral perfusion pressure is the perfusion pressure across the brain and is determined as the difference between the MAP and the ICP: CPP = MAP – ICP (Table 11.1). The optimal CPP is between 50 and 70 mmHg in adults. A CPP of 45–60 mmHg is typically targeted in children, with the lower values in the range targeted in the youngest children.22 In the setting of TBI, CPP should be maintained >40 mmHg in children less than age 5 years23 and >50 mmHg in children aged 6–17 years (Table 11.2).24 Brain perfusion reaches zero when the ICP exceeds the MAP (Table 11.3). Cerebral Blood Flow Cerebral blood flow (CBF) is largely driven by CPP (CBF = CPP/cerebrovascular resistance) (Table 11.1) and can be measured using invasive techniques, including thermal diffusion flowmetry (TD) and laser Doppler (LD) flowmetry. TD is a technique that provides continuous quantitative brain perfusion measurements. This technique uses two thermistors within a probe: a proximal source set at the temperature of surrounding tissue and a distal censor heated 2 degrees Celsius higher. TD takes advantage of the capacity of blood to dissipate heat to quantify CBF in units of mL/100 g/min.25 TD values below 41415 mL/100 g/min have been associated with cerebral vasospasm in adults with aneurysmal subarachnoid hemorrhage.26 In LD flowmetry, a fiberoptic probe is placed onto the brain parenchyma and detects light reflected by red blood cells to derive blood flow velocity. TABLE 11.1 PHYSIOLOGIC PARAMETERS TABLE 11.2 GOAL CEREBRAL PERFUSION PRESSURE FOR CHILDREN WITH TRAUMATIC BRAIN INJURY TABLE 11.3 GOAL MEAN ARTERIAL PRESSURE FOR CHILDREN WITH TRAUMATIC BRAIN INJURY (CHILDREN’S NATIONAL HOSPITAL) Cerebral autoregulation (CA) refers to the maintenance of CBF across a range of MAPs. (Figure 11.3) This is mediated by sympathetic innervation and local tissue factors. A common method of determining CA is by use of the pressure-reactivity index (PRx). The PRx is a model-based index derived from the relationship between ICP and MAP and assumes that changes in intracranial blood flow are driven by changes in cerebrovascular motor responses.27 Because the PRx is calculated from a Pearson linear coefficient between ICP and MAP, it is reported with values between −1 and +1. A positive result is postulated to represent a passive, nonreactive cerebral vascular bed, while a negative result represents a normally reactive vascular bed. When PRx is plotted using an error bar over a range of CPP, an “optimal cerebral perfusion pressure” can be formulated, representing the threshold above which CPP must be maintained for a patient to be in an optimal autoregulatory state.28 PbtO2 Brain tissue oxygenation is measured as the partial pressure of O2 in brain tissue using either a polarographic method or a measure of oxygen diffusion across a semipermeable membrane. PbtO2 has been used primarily in patients with traumatic brain injury. In adults, a PbtO2 monitor is typically used in conjunction with an ICP monitor and cerebral microdialysis catheter. The recent BOOST-II trial showed that in adults with TBI, there was a significant reduction in the burden of cerebral hypoxia and a trend toward improved functional outcome with therapy guided by PbtO2 and ICP monitoring as compared to ICP monitoring alone.29 The 2019 Brain Trauma Foundation guidelines recommend a minimum PbtO2 of 10 mmHg in children.23 Normal values range from 25 to 35 mmHg but may be regional, withPbtO2 in normal white matter measuring ~20 mmHg and PbtO2 in normal gray matter measuring 35–40 mmHg. Cerebral autoregulation can also be measured using PbtO2 monitoring and the oxygen reactivity index, a model-based index evaluating the relationship between PbtO2 and MAP.30 Microdialysis Cerebral microdialysis is a real-time measurement of cerebral interstitial space extracellular fluid analyte concentrations, made using a dialysis catheter with a semipermeable membrane that is typically placed in the white matter. The usual analytes measured are glucose, pyruvate, lactate, glycerol, and glutamate (Table 11.4). The term metabolic crisis has been applied to elevations in the lactate/pyruvate ratio (LPR). Studies in pediatric patients with TBI demonstrate an association between anaerobic metabolites and poor outcome.31–33 Depth Electrodes (DE) Intracranial EEG is now being used in acute brain injury, including in patients with TBI and SAH. Electrographic seizures are seen with DE that are not detectable on scalp recordings.34 The DE should be placed in the tissue at maximal risk of secondary neurologic injury, including seizure activity. MMM, including DE, is now considered standard monitoring for TBI and SAH at several large adult centers. Waziri et al. placed an 8-contact DE in 16 patients undergoing invasive monitoring after acute brain injury, 14 of whom had cEEG available for comparison.34 Clinically important findings were detected in 12 of the 14 patients 415with both depth electrodes and conventional EEG, including electrographic seizures in ten and acute changes related to secondary neurologic injury in two, one with ischemia and one with hemorrhage. In the ten patients with electrographic seizures detected on DE, no surface EEG correlate was seen in six, and rhythmic delta activity was seen in two. In the remaining two patients, electrographic seizure activity was detected on scalp EEG but only intermittently relative to the seizure activity detected by the depth electrodes. In the two patients with secondary injury, an attenuation or suppression burst pattern was seen on depth EEG 2–6 h before changes in other monitoring parameters, including scalp EEG, and 8 h before clinical deterioration. This mirrors the change in the alpha-delta ratio detected by qEEG monitoring that is seen hours before clinical signs of delayed cerebral ischemia in patients with subarachnoid hemorrhage.35–37 In a follow-up multicenter study, Vespa et al. reported seizures or periodic discharges in 62% of patients with severe TBI; these were detected by invasive electrodes but not scalp EEG in 43%.38 Of note, metabolic crisis detected by microdialysis was more severe when epileptiform activity was present on the scalp and/or depth EEG. TABLE 11.4 CEREBRAL MICRODIALYSIS In children, Appavu et al. performed intracranial EEG in 11 children with severe TBI using a 6-contact DE in conjunction with surface cEEG. Four patients had epileptiform activity on DE, two of whom had epileptiform abnormalities noted only on DE, and one patient had seizures detected on DE that were seen before they were detectable on surface EEG. Epileptiform abnormalities were associated with stroke or malignant cerebral edema (Figure 11.4).39 Intracranial EEG can also be used to detect cortical spreading depressions (CSDs), transient and terminal cortical depolarizations.40 These EEG waveforms can be seen on conventional surface EEG or invasive EEG monitoring, including subdural grids, strips, or DE. In addition to the above modalities, neuroimaging is included as part of multimodality monitoring, as it can be used to assess brain anatomy and function and has been correlated with other MMM techniques. In comparison to the techniques described above, neuroimaging has poor temporal resolution.41 To date, in children, MMM has been applied to the treatment of severe traumatic brain injury (TBI)42,43 (Figures 11.5 and 11.6). Continuous EEG Monitoring The EEG is comprised of activity of various frequencies that depend on CBF.44 Normal CBF is approximately 50 mL/100 g/min. As CBF decreases in the setting of ischemia, EEG changes occur: as CBF decreases to 25–35 mL/100 g/min, there is a loss of faster frequencies; at 18–25 mL/100 g/min, there is increased slowing (4–7 Hz), which may be rhythmic; at 12–18 mL/100 g/min, there is a further increase in slowing (1–4 Hz); and at <10–12 mL/100 g/min, there is EEG suppression and loss of electrocerebral activity.44 Therefore, sequential changes in EEG can be used to indirectly indicate a decrease in CBF. Changes in EEG can also be used to identify other types of neurologic deterioration and thereby permit earlier intervention. New-onset seizures could signify a change, such as a new intracranial hemorrhage or infarction. The occurrence of new-onset spikes or sharp waves or a new rhythmic or periodic pattern could also indicate neurologic deterioration or the accumulation of new injury. A sudden change in the EEG background, especially if lateralized or focal, could indicate a new lesion.44 Changes in the EEG background can be especially important in the patient with increased ICP, in whom changes in the EEG, such as the EEG suppression seen during a plateau wave46,47 or with increasing intracranial pressure,48 may occur before a clinically detectable deterioration.48,49 The disappearance of a specific pattern, such as generalized periodic discharges with triphasic morphology, has also been observed in the setting of intracranial hypertension, and these have reappeared when the elevation in intracranial pressure is reversed.45 In general, worsening of the EEG background carries a poor prognosis50 (Figure 11.7). The EEG changes in our ICU monitoring program in the clinical neurophysiology laboratory at Texas Children’s Hospital considered “actionable” are included in Table 11.5. When “actionable” changes are detected by our monitoring team, the clinical teams are immediately notified (Figure 11.8). The Ictal-Interictal Continuum (IIC) EEG waveforms (patterns) may be ictal, indicative of overt electrographic seizure activity, or interictal, indicative of a nonseizure state. cEEG monitoring has identified EEG waveforms that are not clearly ictal or interictal and that have a less certain significance than overt electrographic seizure activity. These waveforms are referred to as the ictal-interictal continuum (IIC) (Table 11.6). Waveforms on the IIC are characteristically rhythmic or periodic.51–54 The IIC is a dynamic pathophysiological state of neuronal instability that suggests an increased risk of evolution to an ictal state and a potential for further neuronal injury. The therapeutic question arises as to whether IIC waveforms indicate the potential for secondary neuronal injury, which would mandate aggressive therapy, or whether they represent an epiphenomenon, arising because of the insult and not causing ongoing neuronal injury, or could they be both? Aggressive therapy should be considered when clinical deterioration is associated with the onset of an IIC pattern. 416TABLE 11.5 ACTIONABLE EEG CHANGES (TEXAS CHILDREN’S HOSPITAL) • Seizures • Spikes • Fast frequencies • Slowing • Discontinuity • Attenuation • Alpha-delta ratio (ADR) Seizures and Status Epilepticus In order to identify IIC waveforms, it is important to first understand the characteristics of electrographic seizures, status epilepticus, and the post-ictal state. An electrographic seizure is an EEG pattern, lasting for a minimum of 10 s, during which the waveforms have the following characteristics: an incrementing onset (increase in voltage and change in frequency) or evolution in pattern; a change in frequency > 1 Hz or change in location; or a decrementing termination (either voltage or frequency).55,56 TABLE 11.6 EEG patterns on the ictal-interictal continuum
Physiologic parameter
Calculation
Mean arterial pressure
1/3 (systolic blood pressure) + 2/3 (diastolic blood pressure)
Cerebral perfusion pressure (CPP)
Mean arterial pressure (MAP) − intracranial pressure (ICP)
Cerebral blood flow (CBF)
CPP / cerebrovascular resistance
Age
CPP goal
0–5 years
> 40 mmHg
6–17 years
> 50 mmHg
Age
MAP goal
Neonate
45 mmHg
1–2 years
56 mmHg
3–4 years
63 mmHg
5–6 years
68 mmHg
7–8 years
71 mmHg
9–10 years
74 mmHg
11–12 years
76 mmHg
13–14 years
78 mmHg
>14 years
82 mmHg
Analyte
Function of analyte
Change in acute brain injury
Glucose
Fuel source of neurons; converted to pyruvate during glycolysis
Decreases in the setting of reduced perfusion or increased cellular uptake
Pyruvate
Converted to acetyl-CoA during aerobic metabolism; converted to lactate during anaerobic metabolism
Decreases in the setting of decreased oxygen delivery; may increase in the setting of increased oxygen consumption or mitochondrial dysfunction
Lactate
By-product of anaerobic metabolism
Increases during anaerobic metabolism
Glycerol
Lipid component of neuron
Increases in the setting of neuronal injury
Glutamate
Excitatory neurotransmitter
Increases in the setting of neuronal injury
Category of abnormality
Type of abnormality
Epileptiform abnormalities
New-onset seizures
Worsening nonconvulsive seizures
New seizure type, i.e. new seizure location
New onset spikes
Increasing frequency of spikes
Spreading of spikes to a new location
Onset of spikes in a new location
Background abnormalities
Loss of fast frequencies
Loss of alpha activity
New slowing
Worsening slowing
New location of slowing
New discontinuity
Worsening discontinuity
New attenuation
Regional attenuation without delta (RAWOD)
Decrease in ADR
Stay updated, free articles. Join our Telegram channel
Full access? Get Clinical Tree
Multimodal Monitoring and the Ictal-Interictal Continuum
James J. Riviello, Jr. and Brian Appavu
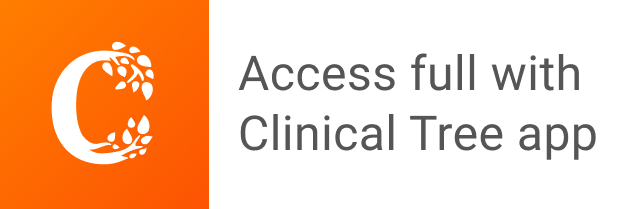