A 34-year-old right-handed woman with a history of smoking presents with a sudden onset of severe occipital headache followed by loss of consciousness that started while cleaning her bathroom. In the emergency department she is found to be arousable to deep stimulation, her pupils are poorly reactive at a 3-mm diameter, and she is withdrawing to painful stimulation bilaterally. When her mental status further decline she is intubated for airway protection. Head computed tomograpy (CT) scanning (Figure 16-1) reveals a subarachnoid hemorrhage (SAH), with thick blood filling the basal cisterns, hydrocephalus, and bilateral intraventricular hemorrhage (IVH). CT angiography reveals an aneurysm of the anterior communicating artery (ACoM). She is transferred to the nearest tertiary medical care center.
Cerebral angiography reveals an 8 × 4-mm ACoM aneurysm that is coiled on SAH day 1 (Figure 16-2). Additionally, angiography reveals severe, bilateral anterior cerebral artery vasospasm that improves after treatment with 12 mg of intraarterial (IA) verapamil. The postprocedural CT scan reveals global cerebral edema and increasing evidence for hydrocephalus. An external ventricular drainage (EVD) catheter is placed. Postoperatively, the patient is found to be in coma with intact brain stem reflexes, bilateral posturing to painful stimulation, and bilateral positive Babinski signs. At that time, the treating physicians decides to place a multimodality neuromonitoring bundle through a right frontal burr whole consisting of a parenchymal intracranial pressure (ICP) monitor, a brain tissue oxygenation probe, and an MD catheter.
One of the most important goals of neurologic critical care is to detect secondary brain injury at a time when permanent damage can still be prevented. The clinical examination remains the gold standard for the assessment of patients with neurologic disease despite great advances in neuroimaging and other diagnostic tools. Furthermore, in medical intensive care unit (ICU) patients daily interruption of sedation has been shown to decrease duration of mechanical ventilation, shorten hospital stay, and in combination with spontaneous breathing trials lead to improved outcome.1,2 There is some evidence suggesting that daily interruption of sedation is safe even in patients with brain injury,3 but this remains controversial.4 Neurologic wake-up trials have been associated with a cardiorespiratory stress response, increased stress hormone levels, and episodes of raised ICP > 20 mm Hg in patients with acute brain injury.3,5,6 Multimodal neuromonitoring techniques may allow the interruption of sedation safely by identifying patients in whom sedation holidays are associated with altered brain metabolism or brain tissue hypoxia.7 Clearly there are a number of patients with acute brain injury in whom interruption of sedation is contraindicated, such as those with ongoing status epilepticus, severe ICP crises, or respiratory failure requiring sedation, as well as possibly paralysis.
Comatose and sedated patients with brain injury are often compared to a “black box” when assessing the neurologic status. Using clinical parameters alone, it may be difficult to differentiate changes in brain physiology that warrant interventions from medication effects. Clinical examinations lack sensitivity to detect some secondary complications such as nonconvulsive status epilepticus (NCSE)8 or silent infarction.9 Inter- and intrarater reliability may be a factor to consider depending on the skill of the examiner.10 Commonly used clinical scales may be too crude to detect secondary complications. The most widely used clinical scale in the ICU environment is the Glasgow Coma Scale,11 which was developed to facilitate rapid communication between first responders and specialized brain injury units to triage patients with traumatic brain injury (TBI). This widely used scale does not reliably detect secondary complications and has been shown in some studies to have poor predictive power for outcome.12 More recently, modifications such as the FOUR score have been proposed,13 but likely these will not be adequate monitors to detect secondary injury in brain-injured patients.14 Invasive monitoring of the brain has the advantage of allowing real-time continuous assessment of brain physiology and has been shown to be safe.15–19 Additionally, as outlined below neuromonitoring may be used to quantify the effect of interventions and prognosticate the outcome. It is important to mention that no single monitoring device will improve outcome per se, unless changes in physiologic variables are coupled with effective therapeutic interventions.20
The most established brain monitoring technique to assess ICP, which can be accomplished with probes placed in the ventricle, brain parenchyma, the subarachnoid space, or subdural space. Currently probes are placed almost exclusively into the ventricular or brain parenchyma, given the risks and inaccuracy associated with other probe locations.21 The presence of hydrocephalus commonly triggers the use of EVDs in patients with SAH for both drainage and at least intermittent ICP measurements. There is no consensus about the duration of EVD clamping time in order to retrieve accurate ICP measurements, and a few new ventricular drainage catheters allow simultaneous drainage and continuous ICP measurements. ICP measured from EVDs are more of a global measure as pressure anywhere on the ventricular system is reflected. Intraparenchymal probes, on the other hand, allow continuous measurements of ICP in the compartment within they are placed in. Depending on the goal of monitoring, more localized or global measures should be chosen. It is recommended to measure mean arterial pressure (MAP) at the height of foramen monro; however, a larger heterogeneity in clinical practice is still evident.22
Measuring ICP allows calculation of cerebral perfusion pressure (CPP) using the simple formula: CPP = MAP – ICP. Importantly, the CPP reflects an assessment of the pressure gradient, which should not be confused with the amount of blood flow to the brain, called the cerebral blood flow (CBF), or an assessment of brain metabolism or oxygenation status (partial pressure of oxygen in brain tissue [Pbto2]). Currently, no consensus exists regarding the selection of monitoring devices for specific patient groups. The selection of devices needs to be made based on the questions that the clinician wants to have answered for a specific patient and based on local experience with monitoring devices (Table 16-1). Findings from just one device may be more difficult to interpret than a more complete assessment that quantifies pressure, metabolism, and oxygenation status at the same time. Additionally CBF monitors and intracranial electroencephalographic (EEG) monitoring are being used in some centers.23–25 Bedside monitoring facilitates immediate detection of changing brain physiology and allows targeted treatment before clinically apparent and often irreversible clinical deterioration occurs.26 Ideally, neuromonitoring should be continuous, time synchronized and available in a simple plotted version to observe trends and indicate clinical relevant events.20 However, the use of invasive neuromonitoring remains controversial.17,27–29
Monitored Phenomenon or Physiology | High Spatial Resolution | High Temporal Resolution |
---|---|---|
Intracranial pressure | na | Pressure monitors inserted into the ventricle, brain parenchyma, subarachnoid or subdural space |
Neuronal injury | Imaging techniques (ie, apparent diffusion coefficient or diffusion tensor imaging on MRI) | Microdialysis (ie, glycerol) |
Large white matter tracts | MRI diffusion tensor imaging, evoked potentials | Continuous evoked potentials |
Large blood vessels | Digital subtraction, MR or CT angiography, Duplex | Continuous Duplex |
Flow velocity in blood vessels | TCD (large vessels) | Continuous TCD |
Brain perfusion | MR or CT perfusion, arterial spin labeling, SPECT, PET | Thermal diffusion based micro-catheter, near infrared spectroscopy |
Neuronal activity and function | Functional MRI, resting state MRI | cEEG, intracortical EEG |
Tissue oxygenation | PET | Clark electrode measuring partial brain tissue oxygenation, near infrared spectroscopy |
Cerebral metabolism | PET, MR spectroscopy, resting state MRI | Microdialysis, difference calculated between arterial and jugular venous oxygenation |
In this patient we selected ICP monitoring because of the high risk for intracranial hypertension with both EVD catheter and parenchymal catheter). Additionally, we initiated brain oxygen tension (Pbto2) and neurochemical monitoring (cerebral MD) because of the high risk of secondary complications in this patient, which include ischemia from vasospasm.
All monitoring devices discussed above measure local brain physiology. Knowledge about catheter location is crucial for the treating neurointensivist in order to correctly interpret the collected data. Currently, no consensus exists regarding the desired placement of devices in relation to injured brain tissue. Generally, most practitioners recommend placement in tissue at highest risk for secondary complications. Ipsilateral ICP monitoring is preferred when the goal is to reduce ICP in the injured hemisphere.21 In patients with spontaneous SAH, the tissues at highest risk for vasospasm and delayed cerebral ischemia (DCI) are those in the area perfused by the ruptured artery.30 However, vasospasm is a diffuse pathology, and delayed ischemia may occur in a distant territory. Therefore, local invasive neuromonitoring may miss the onset of DCI.31 In patients with focal brain injury such as intracranial hemorrhage (ICH), cerebral contusion, or a well-demarked area of hypodensity in a vascular distribution consistent with an infarct peri-lesional placement, placement is generally attempted. Currently used placement techniques at the bedside through a burrhole are not very accurate in reaching this goal. Placement of neuromonitoring devices inside the lesion, ie, within an infarct or hemorrhage will not yield any meaningful data.16 Using a CT-guided neuro-navigated approach may allow for more accurate perilesional catheter placement. In patients with diffuse or nonfocal injury (diffuse SAH, multifocal bilateral injuries, global cerebral edema, or confined IVH) the neuromonitoring devices are generally placed in the frontal lobe of the nondominant hemisphere. A CT scan after neuromonitoring device placement is recommended to determine probe location and to detect procedure-related complications such as bleeding. Neuromonitoring data (ie, Pbto2, brain metabolism) should be interpreted once a postinsertion CT scan has verified probe position. Probe location influences absolute values and responses to therapeutic interventions and its association with outcome.15 Therefore, interpretation of trends rather than absolute values is recommended to detect early changes in brain physiology that may lead to secondary brain injury.
In the absence of large multicenter trials no exact numbers are known, but the most significant complications include intracerebral, subdural, or epidural bleeding; encephalitis or meningitis; and device malfunctioning or misplacement.32 Major bleeding risk is higher in patients who receive antiplatelet agents such as clopidrogel or aspirin. Extreme caution is warranted in those receiving antiplatelet agents and those with a low platelet count or dysfunctional platelets (ie, during uremia). Most series report an infection risk of ≤ 5%,32,33 whereas antibiotic prophylaxis remains controversial and is not generally recommended.32–34 Technical complications (ie, malfunctioning, dislocation) may be up to 14%, especially in the early phase of implementation. Neuromonitoring data may not be accurate in the first hours due to insertion trauma and adaptation issues (ie, for brain metabolism and Pbto2). It is recommended to wait for at least 2 hours to retrieve accurate data.
Recently, the Neurocritical Care Society and the European Society of Intensive Care Medicine promoted a multidisciplinary, multinational panel aimed to evaluate the available evidence and give practical recommendations for bedside multimodality monitoring. A systematic review of the existing literature until 2013 was performed. Monitoring brain oxygen was recommended in patients with or at risk of cerebral ischemia and/or hypoxia, using brain tissue (Pbto2) or/and jugular venous bulb oximetry (jugular venous oxygen saturation [Sjvo2]).20 The quality of evidence is low because limited data are available and a prospective randomized controlled trial is still outstanding. Guidelines from the Brain Trauma Foundation recommend monitoring of Pbto2 or Sjvo2 if hyperventilation is used (level III).35 Further, it is advised to address Pbto2 levels < 15 mm Hg with appropriate treatment strategies (level III).36 For other diagnoses there are no recommendations to be found. SAH guidelines published by the American Heart Association and the European Stroke Organization Federation of Neurological Societies do not address the issue of Pbto2 measurement.37–39
Pbto2 is a marker of the balance between oxygen delivery and oxygen consumption in brain cells and is assessed using a Clark electrode that samples the tension of oxygen in the extracellular fluid reflecting a small volume of brain (17 mm3) at the distal end of the probe. A recently introduced parenchymal oxygen monitor applies an optical principle with light being quenched by molecular oxygen. Comparative studies show differences in absolute Pbto2 values and differences in response to increase in FIo2 (fraction of inspired oxygen) or MAP.40
The health of the brain tissue that is monitored with the probe significantly correlates with the behavior of the brain oxygen measurements.41,42 In healthy normal brain, Pbto2 is 25 to 35 mm Hg at all times, and there is a good correlation between CPP and Pbto2 when Pbto2 is low, suggesting intact autoregulation. In dead brain tissue, Pbto2 levels are generally < 5 mm Hg, and no correlation with CPP is observed. If the probe is placed in the penumbra, Pbto2 may be low, and there is a general correlation with CPP, suggesting disturbed autoregulation.41,42 Although CBF and CPP are important determinants of Pbto2, Pbto2 cannot be regarded as a simple monitor of ischemia as many other variables (ie, partial pressure of carbon dioxide, arterial [Paco2], cerebral metabolic rate, oxygen diffusion, and oxygen extraction fraction).15
A number of studies found a good correlation between a low Pbto2 (< 15 mm Hg) and poor outcome after SAH43 and in patients with TBI.17,42,44–48 The association with mortality, and neuropsychological deficits supports the additional information of this neuromonitoring tool in patients with TBI and SAH.15 Probe location is an important determinant in data interpretation and prognostication. In a retrospective analysis of 405 patients with TBI the prognostic information from the Pbto2 values was available only in patients in whom the probe was located in abnormal brain tissue.49
However, from these data it is not clear if the low Pbto2 is just a surrogate marker for the extent of brain injury or a modifiable measure that can serve as a treatment target. The idea behind cerebral oxygen monitoring is to address these changes to hypoxic values with appropriate treatment strategies. Pbto2-guided therapy to prevent hypoxic brain injury has been associated with improved outcome in uncontrolled, single-center trials.29,50,51 Based on these findings, a multicenter randomized controlled trial in patients with severe TBI (GCS ≤ 8) is underway. (The phase II trial titled “Randomized Controlled Trial of Brain Tissue Oxygenation Monitoring” is funded by the National Institute of Neurological Disorders and Stroke.)
In our patient the bundle was placed into the right frontal white matter. Biochemical analyses revealed lactate to pyruvate ratio (LPR) values ranging between 22 and 29 and brain glucose in the range of 0.8 to 1.2 mmol/L. Pbto2 was 22 mm Hg. The patient developed ventilator-associated pneumonia, which was treated with aminopenicillin. Clinical examination showed improvement with normal brain stem reflexes and withdrawal responses to painful stimulation. Routine transcranial Doppler (TCD) sonography revealed normal velocity in anterior and posterior circulation. On day 5, Pbto2 dropped to 10 mm Hg without a change in brain metabolism or the clinical examination.
Pbto2 measures the balance between oxygen delivery and oxygen consumption in brain cells and may be influenced by a host of different local factors including oxygen consumption of neurons and glia, oxygen diffusion conditions and gradients in the sampled brain tissue, the number of perfused capillaries per tissue volume, the length and diameter of perfused capillaries, the capillary perfusion rate and microflow pattern, and the hemoglobin oxygen release in the microcirculation. Systemic factors that may influence Pbto2 measurements include ICP, arterial blood pressure partial pressure of oxygen, arterial [Pao2] and Paco2, pH, temperature, blood viscosity, and hematocrit.
A recent study suggests that local brain tissue oxygen tension is more closely related to CBF and the diffusion of dissolved plasma oxygen rather than total oxygen delivery to the brain and oxygen metabolism of the brain cell.52 Strategies to influence low Pbto2 are multiple and should be determined on an individual basis (Figure 16-3).53,54 Although Pbto2 may increase in one patient after CPP optimization or improvement of cardiac function, other patients may benefit from an increase in oxygen transport capacity (blood transfusion). Additional monitoring devices (CBF, brain temperature, EEG) may be helpful for the clinician to narrow down the differential diagnosis of a low Pbto2.
Low values may be observed without changes in brain metabolism as this strongly depends on the duration of brain hypoxia. Basic strategies to minimize oxygen consumption of the brain include aggressive fever management, treatment of shivering,55,56 management of pain and agitation (sedation and adequate analgesia), and optimization of positioning of the upper part of the body (elevated 30°).
The next questions use a correlational data analysis approach to better understand brain physiology in the acute brain injury setting, which may help clinicians to understand complex physiologic relationships and identify optimal physiologic targets in real time. Additionally, the behavior of physiologic parameters in relation to interventions (eg, increasing MAP/CPP, optimizing cardiac output, decreasing ICP, blood transfusion, and ventilator strategies) may identify individualized thresholds such as MAP goals and assess cerebral autoregulation.
In general there is a close relationship between and Paco2 as well as end-tidal CO2 (Figure 16-4). Hyperventilation leads to cerebral vasoconstriction and may cause infarction. In TBI patients, hyperventilation is only recommended as a temporizing measure for increased ICP (level III) and should not be used in the first 24 hours after head injury (level III).35 Prophylactic hyperventilation is not recommended (level II), and if hyperventilation is used, Sjvo2 or Pbto2 measurements are recommended to monitor oxygen delivery (level III).35
In our patient we chose a CO2 target of 30 to 33 mm Hg using end-tidal CO2 measurements.
Figure 16-4.
Correlation between end-tidal CO2 and Pbto2 in our patient. Monitoring of CO2 is crucial even in awake patients after severe brain injury. Despite these recommendations, a recent survey conducted in Europe showed that prophylactic hyperventilation (30-35 mm Hg) is still commonly used after severe TBI.57 Additionally, these authors found that Pbto2 or Sjvo2 monitoring are rarely implemented even if patients were hyperventilated to a level < 30 mm Hg. A low CO2 level is associated with tissue hypoxia after severe head injury, and unintentional spontaneous hyperventilation may be a common and under-recognized cause of brain tissue hypoxia after severe brain injury.58,59

Figure 16-5 illustrates the tight correlation between CPP and Pbto2 in our patient during a 24-hour monitoring period. Brain tissue hypoxia (< 15 mm Hg) is observed at CPP values of ≤ 90 mm Hg. There are several treatment options to optimize CPP in this patient. As a first step, the intravascular volume status should be optimized, which may be estimated using a clinical assessment or monitored parameters such as the stroke volume variation, the global end-diastolic index, or the pulmonary artery wedge pressure. If optimizing volume status does not result in improved CPP and Pbto2 status, MAP may be titrated up to achieve Pbto2 improvements > 20 mm Hg. Depending on cardiac performance (which can be estimated using the cardiac index or the ejection fraction), this can be achieved using pressors, including norepinephrine, phenylephrine, milrinione, dobutamine, or dopamine.
In our patient the global end-diastolic index (GEDI) was elevated at 800 after volume resuscitation, and levophed was used to increase MAP. After optimizing these physiologic parameters, no further episodes of brain hypoxia were seen (Figure 16-6).
This case illustrates how Pbto2-guided therapy may lead to more aggressive management. Additionally, hypoxic and ischemic events can also be observed despite maintaining a CPP > 70 mm Hg in patients with severe brain injury. A deescalation strategy with lowering CPP may improve brain oxygenation; however, once the CPP reaches the lower threshold of the autoregulatory breakthrough zone, hyperemia and a secondary increase in ICP may result (Figure 16-7).
Increasing FIo2 effectively increases local Pbto2. However, this does not necessarily lead to an improvement in brain metabolism. What has been described is a simultaneous decrease in lactate and pyruvate, leaving the LPR overall unchanged. This would suggest that hyperoxia may lead to retardation of the metabolism rather than a stimulation.60 Another study showed that hyperoxia may decrease brain lactate levels.61 Contrarily, hyperbaric hyperoxygenation may have a positive effect on Pbto2 and brain metabolism.62
Our patient’s hemoglobin was 8.5 mg/dL, with a Pbto2 level < 12 mm Hg. Should this patient be transfused?
Blood transfusions increase oxygen transport capacity to the brain and have been shown to increase Pbto2 after severe head injury without improving brain metabolism.63 In a randomized controlled trial a transfusion threshold of < 7 mg/dL was not associated with increased 30-day mortality in critically ill patients.64 For patients in the NeuroICU, there is conflicting evidence about the optimal transfusion threshold. Decisions regarding blood transfusions in patients with SAH must be individualized because optimal transfusion triggers are not known (level III).65 Currently there are a number of trials under way to compare different transfusion thresholds for patients with SAH.65–70 Subpopulations of patients with SAH, such as those with ongoing delayed cerebral ischemia from vasospasm, may benefit from higher transfusion thresholds (ie, keeping hemoglobin > 10 mg/dL).69,71 Patients with moderate-to-severe TBI do not benefit from a “liberal” transfusion strategy (level II).65 Recent reports on multimodal neuromonitoring and hemoglobin suggest that a low hemoglobin level is associated with brain metabolic distress (LPR > 40) and low cerebral glucose concentration (< 0.7 mmol/L).63,72 Anemia, defined as hemoglobin ≤ 9 mg/dL, was not associated with poor outcome in 80 patients with severe TBI.73 However, simultaneous occurrence of anemia and compromised Pbto2 was associated with poor outcome. Integration of multimodal neuromonitoring data may help to identify patients who may benefit from a specific intervention. At this point, data are too preliminary to define optimal transfusion thresholds. However, the assessment of brain physiology using invasive multimodality monitoring may support decisions for a transfusion threshold in selected individuals.
In patients with ICP elevation, an aggressive stepwise approach to lower ICP is indicated. Lowering ICP is usually performed in stepwise fashion; however, several treatments may and should overlap or may be individually applied in a different order. Some of the measures used include cerebrospinal fluid (CSF) diversion by EVD, sedation, and analgesia, surgical decompression, short-term hyperventilation, osmotic agents, therapeutic hypothermia, and EEG-guided barbiturate coma.
Osmotherapeutic agents include mannitol (1-1.5 g/kg IV, bag-infused over 30 minutes, q6h; avoid serum Osm > 360 mosm/L and Osm gap > 10) and hypertonic saline 23.4% (30 mL over 5 minutes IV, q4-6h; avoid serum Na > 160 mg/dL). Both osmotherapeutics effectively decrease ICP. Recent studies suggest that hypertonic saline, but not mannitol may increase Pbto274,75 (Figure 16-8 and 16-9), mostly through improvement of cardiac function and secondary increase of CPP; however, mannitol may improve brain metabolism.76 So far, no treatment can specifically be recommended unless one drug is relatively contraindicated (Figure 16-10).
Figure 16-9.
Line graph illustrating mean (SD) partial pressure of oxygen in brain tissue (Pbto2) at baseline (time 0) and at 30, 60, and 120 minute after hypertonic saline and mannitol bolus administration. *P, 0.05, **P, 0.01 for comparisons between the two treatments. (Reproduced with permission from Oddo M, Levine JM, Frangos S, et al. Effect of mannitol and hypertonic saline on cerebral oxygenation in patients with severe TBI and refractory intracranial hypertension. J Neurol Neurosurg Psychiatry. 2009;80(8):916-920.)74

Figure 16-10.
The average time course of lactate to pyruvate ratio (LPR), intracranial pressure (ICP), and brain tissue oxygen pressure before (−180 minutes to time 0) at (time = 0) and after (0-240 minutes) mannitol 20% infusion. Error bars, mean ± 1 SE of N = 22 individual trials. (Reproduced with permission from Helbok R, Kurtz P, Schmidt JM, et al. Effect of mannitol on brain metabolism and tissue oxygenation in severe hemorrhagic stroke. J Neurol Neurosurg Psychiatry. 2011;82(4):378-383. With permission from BMJ Publishing Group Ltd.)76

Does optimization of cardiac performance in patients with normal ICP improve oxygenation status?
Yes, it may. As shown in Figure 16-11 cerebral oxygenation, as measured by Pbto2, may improve with increased cardiac output. CO can be optimized with the use of inotropic agents in volume-resuscitated patients with inadequate cardiac output or through the infusion of fluids in preload-responsive patients. Improvement in cardiac index after fluid resuscitation was associated with improved oxygen delivery in patients with SAH.77 In patients with inadequate oxygen delivery to the brain despite normal ICP and CPP, the improvement in cardiac performance may further optimize CBF and potentially improve aerobic metabolism.
Can a correlational analysis of multimodality parameters be used to assess autoregulatory status?
Yes. It has been known for more than a decade that there is a relationship between the autoregulatory status of a patient regarding the arterial BP vs ICP correlation,78 which has been termed the PRx and is calculated as a moving correlation between BP and ICP. A negative correlation is seen in patients with vasoreactivity, whereas a positive correlation indicates a passive pressure response system and is seen absent vasoreactivity (Figure 16-12).
Figure 16-12.
Pressure reactivity index calculated as the correlation coefficient between arterial and intracranial pressure in a patient with absent autoregulation. (Reproduced with permission from Czosnyka M, Smielewski P, Kirkpatrick P, Laing RJ, Menon D, Pickard JD. Continuous assessment of the cerebral vasomotor reactivity in head injury. Neurosurgery. 1997;41(1):11-17; discussion 17-19. By permission of Oxford University Press.)78
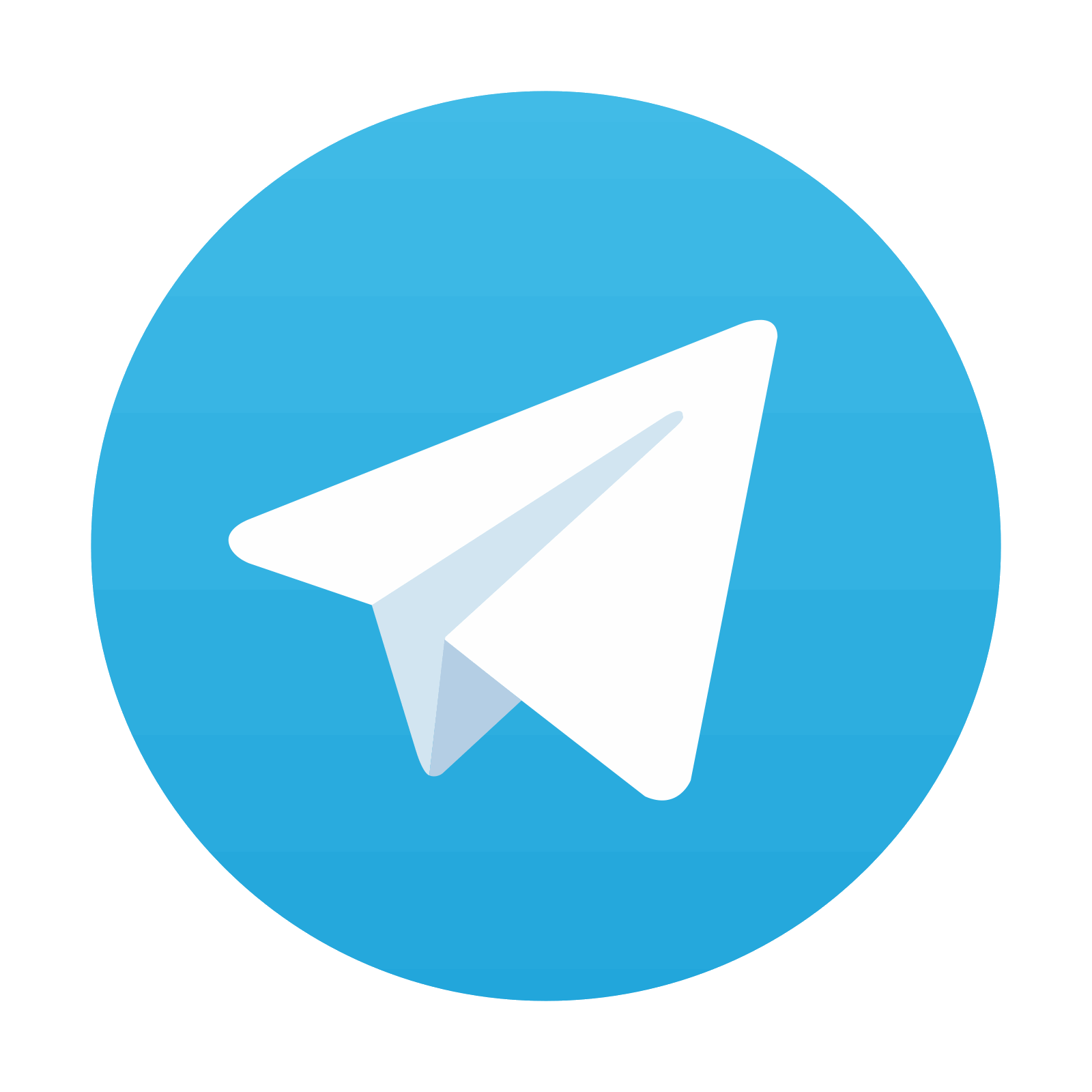
Stay updated, free articles. Join our Telegram channel
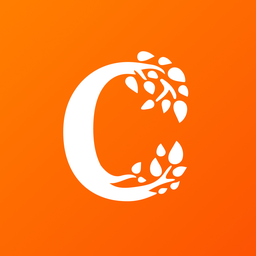
Full access? Get Clinical Tree
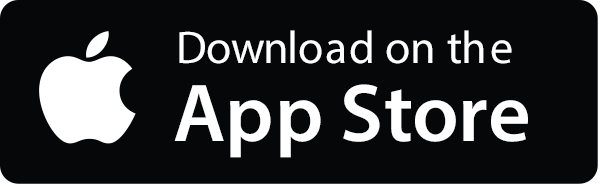
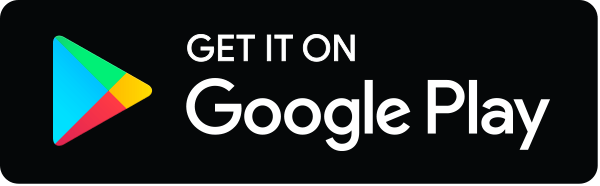
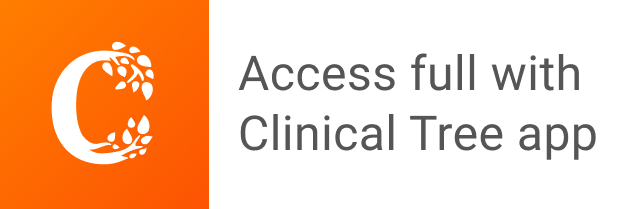