Summary of Key Points
- •
Muscle integrity is integral to both spine form and efficient function.
- •
For most patients with spine-related disease, exercise is not only safe, but it is the necessary component to improved functional outcomes.
- •
Based on the available literature, extension-biased exercises that emphasize strength of the deep posterior musculature consistently show greater benefit than focal abdominal muscle strengthening.
- •
A sagittal and coronally balanced spine with intact curves provides efficient function for the spine and is noted by electromyography silence within normal functional parameters.
- •
Muscle-sparing surgery (minimally invasive) is a promising but unproved innovation that aims to decrease convalescence, decrease surgical morbidity, and improve functional restoration.
The muscles that act as the dynamic control mechanism for the spine constitute the largest collective, coordinated group of muscles in the human body. That preeminence is understandable, as actions of the spine muscles are the key factor that allows humans to be bipedal creatures. Unfortunately, spinal muscles have been inadequately appreciated and regarded (“lift with your legs, not with your back”), incised with impunity, injured from retraction, neglected through rest/traction, and denervated capriciously. Though our knowledge of spine muscle form and function continues to grow, there remains much to be learned, from the best way to rehabilitate these muscles to the optimum surgical technique for muscle preservation. This chapter challenges the reader to understand that functional ability relies on the preservation and rehabilitation of spinal musculature and the maintenance of coordinated spine muscle performance.
Functions of the Back Muscles and Their Fascia
Muscle acts to balance and maintain the integrity of the spine throughout its range of motion. The co-tensioning of abdominal muscles at a distance, combined with the collective dorsal force of the lumbar back muscles during flexion-extension, allows maintenance of a “balance point.” Coupled ventral and dorsal forces have a net compressive force that, in turn, balances motion at the instantaneous axis of rotation for each motion segment—thereby maintaining compression at the disc and minimizing angular change. This net muscle force offsets other forces, thereby maintaining the force vector perpendicular to the disc’s plane and stabilizing the spine, like the guy-wires that allow flimsy tent material to withstand 100 mph winds ( Fig. 6-1 ). The compressive force vector likewise minimizes shear forces, implicated as a leading cause of disc degeneration.

In maintenance of upright posture, the joints and ligaments of the spine are the primary stabilizers, with only about 2% to 5% of maximum isometric back muscle strength utilized in this position. However, the amount of activity in the lumbar back muscles during relaxed standing varies between subjects, showing a low level of constant activity, discontinuous activity, or no activity. Such variety is likely due to variances in the sagittal/coronal “plumb-line” (from skull base to pelvis) between subjects. The gravity line passes in front of the L4 vertebra in approximately 75% of people, exerting a constant flexion moment on the lumbar spine that must be opposed by the lumbar extensors to maintain a relaxed upright posture. When the sagittal gravity line lies posterior to the lumbar spine, abdominal muscle activity is required to maintain the posture. Similarly, postures that shift the coronal gravity line, such as carrying a weight in one hand, result in lateral flexion of the spine. Contralateral lateral flexors are then called to bring the spine to neutral. Sitting activity in these muscles is only slightly greater than relaxed standing, utilizing 3% to 15% of maximal isometric back muscle strength. However, activity in these muscles abolishes or decreases when sitting with arms supported on a table or when leaning forward with elbows supported by knees. Finally, little activity is seen in these muscles with reclined sitting, lessening with a greater reclining angle.
Muscle plays another noteworthy role, the shaping and maintenance of the spinal curves. Spine biomechanical research suggests that the passive tension band from dorsal spinal muscles (with minor hoop stress contributions of abdominal muscles) is the primary generator of the curvilinear structure of the spine. This curvilinear structure enables geometrically greater load bearing than straight-spine models (1200 Newtons versus 100 Newtons). Further, instantaneous, axial-rotational forces between segments in straight-spine models may lead to rapid failure when the spine is progressively loaded.
Deviations from neutral posture that are not resisted utilize a small initial movement from the abdominals, back extensors, or lateral flexors with gravity supplying the force to continue the movement in that direction. However, greater antagonist eccentric muscle activity is necessary to control such movement. So, for example, in leaning backward, abdominal activity is required to arrest or slow motion and to maintain the off-neutral posture. However, if the backward lean is resisted, more recruitment takes place, but only in the long paraspinal muscles that act on the thorax, not, for instance, the multifidus.
The most pronounced function of the lumbar back muscles takes place with flexion and extending from the flexed position. Upon forward flexion, lumbar paraspinal extensor activity increases as the spine flexes further forward or as greater weight is carried during the motion. Again, eccentric contraction of the lumbar extensors controls the speed flexion with eccentric action. The multifidus, lumbar iliocostalis, and lumbar longissimus control flexion of the lumbar spine, and the lumbar iliocostalis and lumbar longissimus control anterior translation of the lumbar vertebrae. Flexion or extension of the thorax on the lumbar spine is similarly controlled by firing of the thoracic portions of iliocostalis and longissimus. During forward flexion a so-called critical point is reached at which the back muscles deactivate or may achieve electromyographic silence and the spine is supported purely by its posterior ligaments and the zygapophyseal joint capsular resistance. This phenomenon is termed the flexion-relaxation phenomenon. It occurs when the spine is at around 90% of full spine flexion or later if a weight is carried, with roughly 40% of hip flexion excursion yet to occur. Extension from the flexed position results in pronounced activation of muscles attached to the erector spinae aponeurosis and multifidus exerting posterior rotation on the vertebrae. When heavy loads are lifted, 75% to 100% of maximum isometric back muscle strength is utilized.
Fascinatingly, humans can demonstrably lift greater weights than allowed by biomechanical calculations of spinal musculature. How such a feat is performed has yet to be determined. In the 1950s, a theory was proposed, and subsequently popularized, stating that increased abdominal pressure exerted by abdominal muscles aided in extending from a flexed position when carrying a load. However, more recent studies and biomechanical analyses have dispelled this notion. Indeed, increased intra-abdominal pressure as caused by a Valsalva maneuver actually raises (does not decrease) the load on disc-joint elements of lumbar spine without necessarily decreasing the neutral zone. The theory that the abdominal muscles (indirectly attached to the lumbar spinous processes via the thoracolumbar fascia) exert added extension force has been similarly refuted. Another model proposed that tension on the posterior ligaments of the lumbar spine would allow the hip extensors to indirectly pull the lumbar spine into extension from flexion. However, analysis of posterior ligamentous strength seems to show that the ligaments are not strong enough to bear the loads required in this theory. Calculations involving passive downward resistance of the back muscles have yet to be verified. Other mechanisms have been proposed, including the hydraulic amplifier effect and models based on arch theory, but confirmation is lacking.
It is also worth noting the importance of hip motion and control with regard to flexion, extension, and load lifting of the lumbar spine. Most forward flexion range of motion involves the hip, rather than the lumbar spine, with hip extensors such as the gluteal muscles and hamstrings controlling descent and animating extension from flexion. The pelvic musculature is also a crucial component of the kinetic chain in force transfer from the lower limbs to the spine and pelvis. These muscles thus play an important, if indirect, role in spinal motion, posture maintenance, and modulation of force transfer through the kinetic chain.
Compressive Loads and Strength of the Back Muscles
Combined disc pressure and electromyographic studies have demonstrated that intradiscal pressure in the lumbar spine increases with increased paraspinal activity. This phenomenon is explained by the great longitudinal forces exerted on vertebral bodies by the paraspinal muscles. Consequently, the greatest rise in disc pressure occurs with forward bending activities, further increasing if trunk weight increases, if a weight is lifted, or if the spine is also axially rotated during flexion. Contrary to popular belief, “lifting with the legs” with a straight back does not significantly change the forces across the discs or activity in the back muscles when compared to stooped lifting, or lumbar flexed lifting. The critical variable in increasing loads across the spine and muscle activity is the distance the weight is held from the body, with greater distance resulting in greater muscle activity and disc pressure.
Back musculature is generally stronger in males than in females and follows a general decline after the age of 30. The muscles attached to the erector spinae aponeurosis provide for 50% of the extension force across the lumbar spine, with the multifidus providing 25% and the lumbar iliocostalis and longissimus providing the remainder. Shear forces are dissipated largely by spine musculature differentially across the lumbar spine, depending on fiber attachment differences and the orientation of various vertebrae with regard to the lumbar lordosis. As such, the upper lumbar disc-vertebral complex sustains posterior shear forces in the upright position, whereas the L5 vertebra sustains an anterior shear force. The force vectors are reversed with complete forward flexion. The variable fiber direction of the deep and superficial muscles redirect shear force to a downward vector by tensioning to decrease the biomechanical neutral zone across each segment.
Histochemistry
Postural maintenance is a long-duration activity, so endurance is critical to back muscle function. Indeed, the back muscles differ from most other human muscles in their greater fatigue resistance. Unsurprisingly, back muscles generally have a higher density of slow-twitch (type I, oxidative type) muscle fibers than other muscles. Specifically, the longissimus has 70% slow-twitch fibers, whereas iliocostalis and multifidus contain 55% slow-twitch fibers. There is a wide range of fatigue resistance, with women exhibiting relatively more slow-twitch fibers and greater paraspinal endurance than men. It would be tempting to presume loss of type I fibers, decreased spinal endurance, and chronic back pain might be connected, but other than the associations of atrophy with decreased isokinetic testing ability, studies thus far have shown mixed results.
Back Muscle as a Source of Pain
It is indisputable that spine muscles can be a source of local and referred pain to the gluteal region, as demonstrated in studies where hypertonic saline was injected into the back muscles of pain-free individuals. Etiologies of muscle pain include physical trauma, ischemia, compartment syndrome, delayed onset muscle soreness, myopathies/myositis, tumor, infection, central causes of spasticity, peripheral nerve trauma, chemical irritation or entrapment resulting in pain in innervated muscles, central sensitization syndromes such as fibromyalgia, and myofascial trigger points/myofascial pain syndrome. A full discourse about pathologic muscle conditions is beyond the scope of this chapter, but some will be considered here.
Myofascial trigger points, as proposed by Travell and Simons, are discrete locations within a taut band of muscle that are actively painful or merely painful to palpation (i.e. latent). Palpation of a trigger point may result in referred pain distal from the site of tenderness and a local twitch response. Trigger points are thought to arise secondarily to overuse of the muscle, local musculoskeletal lesions, postural deficits, and/or central sensitization. However, the existence of myofascial trigger points as intrinsic muscular lesions has been subject to debate and recently to outright refutation by Quintner, who proposed alternative mechanisms for the observed clinical syndrome that involve the central and peripheral nervous systems rather than the muscle itself.
Myotendinous injuries are a consideration with regard to spine pain of muscular origin. Sudden stretch of sufficient force induces limb myotendinous junction failure in animal studies, but similar spine musculature studies have not been done. Furthermore, the presence of such lesions on imaging studies has not been demonstrated.
Muscle spasm induced by sustained postures may be a source of muscle-mediated low back pain. Several feline model studies have demonstrated that sustained lumbar flexion or repetitive lumbar flexion and extension can result in ligamentous laxity and subsequent strong muscle spasms in the lumbar multifidus, iliocostalis, and spinalis muscles. Similarly there is evidence for a reflex paraspinal activation arc with stress to discs, zygapophysial joints, and spinal ligaments stimulating proprioceptive afferents and producing a pronounced reflex activation of paraspinal muscles at the level of the stressed structure but also spanning one to two adjacent segments. Aside from being a source of back pain, muscles are widely thought to be a target intervention to ameliorate spinal pain and reduce the risk for pathology.
Muscle Training to Improve Spinal Stability and Relieve Pain
Panjabi’s influential model of spinal instability proposes two broad categories of spinal instability: gross and clinical or functional . Gross instability involves radiologically visible displacement of a vertebra, caused by significant osseous or ligamentous disruption, and is beyond the purview of this chapter. Given the lack of radiographic findings, the concept of functional instability is difficult to define. Panjabi has defined it as “loss of the spine’s ability to maintain its patterns of displacement under physiologic loads so there is no initial or additional neurologic deficit, no major deformity, and no incapacitating pain.” Pattern of displacement is also referred to as neutral zone, the span of spinal range of motion where active muscular resistance is minimal. In this conception functional instability results from an increase in the neutral zone. Increasing the neutral zone is equivalent to increasing compressive, translational, and shear forces across the joints of the spine, potentially causing pain or accelerating degeneration.
Panjabi proposes three key determinants of spinal stability:
- 1.
Neuromuscular control subsystem (neural elements)
- 2.
Passive subsystem (bony and ligamentous elements)
- 3.
Active subsystem (spinal muscles)
The role of muscle and neuromuscular control in spinal stability cannot be overemphasized. Without spinal and other supportive, so-called core, musculature, the remaining spinal structures would be incapable of even supporting compressive forces equivalent to the weight of the upper body. The spinal ligaments are important components, but most of their restraining capacity is enforced at end range of motion. The neural control mechanism must coordinate proprioceptive sensory inputs with appropriately sequenced activation and deactivation of the active subsystem to meet the functional demands of the spine. Neural control is thus as important as muscular strength and endurance. Finally, the theory purports that each subsystem is capable of making up for deficits in another. This fine balancing act among the three elements of spinal stability can tip over into dysfunction when subtle extrinsic (trauma) or intrinsic (fear-avoidance) disruptions evolve into a feed-forward system of dysfunction. This concept is ably demonstrated by Panjabi’s hypothesis of chronic back pain:
[S]ub-failure injuries of the ligaments and embedded mechanoreceptors … generate corrupted transducer signals, which led to corrupted muscle response patterns produced by the neuromuscular control unit. Muscle coordination and individual muscle force characteristics, i.e. onset, magnitude, and shut-off, are disrupted. This results [sic] in abnormal stresses and strains in the ligaments, mechanoreceptors and muscles, and excessive loading of the facet joints … inherently poor healing of spinal ligaments, accelerate degeneration of disc and facet … [that] over time, may lead to chronic back pain.
The preceding theory underpins the interest in core muscle therapeutic intervention. Strength, endurance, functional range, activation, and coordination deficits in core muscles are thought to predispose people first to functional instability and subsequently to pain and decreasing functional performance. Bergmark theorized a division of core musculature into global and local muscles. The global muscles are the prime drivers of movement with the local muscles providing segmental stabilization and control. Global muscles include the rectus abdominis, external oblique, and thoracic iliocostalis and longissimus. Local muscles comprise deeper components such as lumbar multifidus, psoas major, quadratus lumborum, lumbar iliocostalis and longissimus, transversus abdominis, and posterior fibers of internal oblique. Often included in the definition of core musculature are the diaphragm, pelvic floor, and hip girdle muscles. Electromyographic studies of multifidus and transversus abdominis muscle activation prior to movements of the limbs are thought to support the hypothesis that core strength is the foundation for limb movement. Additionally, pelvic floor muscle co-activation with the transversus abdominis is theorized to provide spinal stabilization and proprioception. Terms used to describe core stability in the therapeutic settings include lumbar/lumbopelvic stabilization, dynamic stabilization, motor control, neuromuscular training, neutral spine control, muscular fusion, trunk stabilization, and core strengthening.
Studies comparing patients with low back pain to controls have revealed findings that lend credence to this theory. Patients with back pain have been shown to have weaker abdominal muscles, delayed activation of the transversus abdominis and multifidus muscles before movements of the limb, hip extensor weakness or strength asymmetry, multifidus atrophy, decreased multifidus endurance relative to other paraspinals, loss of the flexion-relaxation response, and an overall relative decrease in trunk extensor endurance versus trunk flexor endurance. Patients with their first episode of acute back pain have been noted to have multifidus atrophy that does not spontaneously correct after recovery. Findings that demonstrate a learned pattern of “pain inhibition” and physiologic “fear avoidance” may shed light on the mechanism for recurrent low back pain. What has not been conclusively shown is whether low back pain is the cause or result of these changes. The precise cause of reduced strength, endurance, and control in the face of some pain-producing pathology remains underexamined. Such adverse changes undoubtedly occur with prolonged disuse and deconditioning and may precede and predispose to pain. Conversely, the pain itself may inhibit neuromuscular function through a nociceptive reflex feedback mechanism. Also, biopsychosocial phenomena, such as anxiety, fear of reinjury, or depression, may unconsciously attenuate effort or provide a prodrome to reinjury. Whatever the temporal and causal relationship, it has been demonstrated that training can rectify these deficits. For example, training resulted in normalization of transversus abdominis activation in low back pain patients with persistent improvement at 6 months, and a lumbar multifidus exercise program can increase its bulk. Furthermore, electromyography (EMG) biofeedback stretching training can restore the flexion-relaxation phenomenon in chronic low back pain patients, even after spine surgery, resulting in improved flexibility and function. It remains controversial whether positive treatment effects are in fact causally related to improvement in these metrics.
Significant areas of disagreement remain regarding the components of a minimally effective spinal rehabilitation program. Ideally, the program would be tailored to the patient, reassessed throughout, and adjusted appropriately and progressed. A typical core strengthening rehabilitation program consists initially of isometric activation of the multifidus and transversus abdominis followed by static, neutral spine exercises (e.g., planks, side planks, bird dogs, bridges), finally progressing to dynamic, functional, and task-specific exercises outside the neutral spine position that are customized to the patient. Prior expert opinion has warned against progressive resistance strengthening of lumbar extensors, fearing such exercise imparts unsafe loads on the spine. However, a review of uncontrolled studies reported good outcomes with isolated lumbar extension resistance training exercises.
The theoretic basis for motor control exercises in the treatment of low back pain is compelling, but the literature is of mixed quality. The results of systematic reviews of randomized-controlled trials have not shown consensus. Some reviews failed to show superiority of core strengthening exercise over other conservative treatments. One review found motor control exercises to be superior to general exercise or minimal intervention for pain and disability in patients with chronic and recurrent low back pain. The authors have called for the development of standardized clinical assessment methods to allow subclassification of patients’ deficits, informing more personalized, targeted, and possibly more efficacious intervention. Another review found superior short-term, but not long-term, relief of pain in chronic low back pain patients.
The potential benefits of spine muscle training are not merely limited simple pain diagnoses but may include a range of advantages for patients with osteopenia/osteoporosis and vertebral compression fractures. Isometric back extensor exercises may decrease acute vertebral fracture pain. A program of regular gentle resistive back extension exercises using sandbag weights resulted in a significant reduction in vertebral fractures compared to controls at the 10-year follow-up. Back extensor strengthening in patients with osteoporosis was also found to improve activities of daily living, posture, and body shape. However, a Cochrane review of back extensor strengthening exercises for improving outcomes after osteoporosis found inconclusive results, stating that higher-quality studies are needed.
The most important concept about rehabilitation is the consistent failure of symptom-based therapies to show functional improvement in the subacute or chronic back pain population. Starting in the 1980s, numerous authors have demonstrated that “quota-based” rehabilitation programs that gradually escalate this resistance (despite possible increasing pain symptoms) have shown the most consistent results in North America, the Middle East, and Europe.
Anatomy
Psoas Major
The psoas major is a large muscle that spans from the lumbar spine to its insertion in the lesser trochanter of the femur. Its origins are the anterior, medial, inferior portions of the T12 to L5 transverse processes with broad attachments to the midlateral vertebral bodies from T12 to L5 and the intervertebral discs from T12-L1 to L4-5. It is innervated by L2-4 ventral rami via the lumbar plexus.
Its primary action as a hip flexor is confirmed by the morphology of its fiber bundles. Whereas fibers of the psoas are arranged to extend upper lumbar segments and flex lower lumbar segments, its attachments act so close to the lumbar axes of rotation that they contribute very little to lumbar flexion or extension. However, the psoas theoretically acts on the lumbar spine by exerting axial compression forces on the lower lumbar discs. For instance, maximal bilateral psoas contraction would be expected to cause an approximately 100 kg compression load on the L5-S1 disc.
Quadratus Lumborum
This lateral muscle originates at the anterior, medial iliac crest and curiously has significant anatomic variability between specimens. It attaches to inferior rib and medial vertebral body of T12 and the lateral tips of the L1-4 transverse processes with an intricate arrangement of longitudinal and oblique muscle fibers of highly variable sizes. It is innervated by the ventral rami of the T12 to L4 spinal nerves.
Its function is not entirely clear. Its largest and most numerous fibers act from the transverse processes to the ilium, exerting a definite but limited lateral lumbar flexion force. Its fascicular orientation posterior to the lumbar sagittal axes of rotation also allows it to exert downward force and a small extension moment, equivalent to about 10% the extension force of the posterior lumbar extensors.
Lumbar Back Muscles
These muscles lie posterior to the transverse processes. They act across the lumbar spine, whether attached directly to it or spanning across it. Bogduk divides them into three groups:
- 1.
Short intersegmental muscles: interspinales and intertransversarii mediales
- 2.
Polysegmental muscles that attach to the lumbar vertebrae: multifidus, lumbar longissimus, and iliocostalis
- 3.
Long polysegmental muscles that do not attach to the lumbar vertebrae: thoracic longissimus and iliocostalis
Deep Intersegmental Muscles
These small, paired, intersegmental muscles are found throughout the spine. They represent the deepest layer of spinal muscles.
The interspinales extend from the C1 to L5 vertebral bodies and are found between each vertebral level. They attach the lower border of the higher spinous process to the upper border of the spinous process below. These muscles receive segmental innervation from the dorsal rami.
The intertransversarii laterales are small muscles found from L1 to L5, spanning from the inferior transverse process of the upper vertebrae to the superior margin of the transverse process of the lower vertebrae. In contrast to the dorsal spinal musculature, the ventral rami innervate these muscles. They are considered homologues to thoracic intercostal and levator costae muscles.
The intertransversarii mediales extend from the mamillo-accessory ligament and inferior portion of the mamillary process of the vertebrae above to the superior portion of the mammillary process below. Analogous intertransversarii muscles are found throughout the thoracic and cervical spine as well. They are segmentally innervated by the dorsal rami. The obliquely arranged rotatores are also included in this group.
Each individual muscle within this group is very small and thus has limited force generation capability. Additionally the intertransversarii mediales is at mechanical disadvantage due to its location very close to the lateral and sagittal axes of rotation. Whether they offer significant contribution to spinal motion is debatable, but they may primarily serve a proprioceptive function when stretched to guide the eccentric contraction of the larger spinal muscles. This proposition is supported by the finding that all of these small intersegmental muscles of the spine contain a much higher concentration of muscle spindles than the longer polysegmental muscles.
Multifidus
The multifidus is a polysegmental muscle with a complex fascicular arrangement extending throughout the spine from cervical to sacral levels. It is the most medial of the polysegmental spinal muscles. Its various groups of fibers have many overlapping attachments. In the lumbar spine ( Fig. 6-2 ), there are two fiber types, laminar and spinous process fibers. The shorter laminar fibers attach to a posterior, inferior dorsal lamina and the fibers extend obliquely to a mammillary processes two levels below or to an area just above the dorsal S1 foramina in the case of the laminar fibers of the L5 multifidus. The longer fibers attach to the inferior lateral base of a spinous process, or more commonly to the inferior tip of a spinous process by way of a common tendon, and descend two or more levels obliquely to various targets, including a mammillary process, facet joint capsule, medial iliac crest, posterior superior iliac spine (PSIS), and an area just above a dorsal sacral foramen below. In the lumbar spine, the segments are predictably innervated by the medial branch of the dorsal ramus that exits just below the pedicle the most proximal vertebral body to which it attaches (e.g., multifidus fibers attached to the L1 spinous process or lamina are innervated by the medial branch of the L1 dorsal ramus).

The primary action of the multifidus is segmental extension by means of posterior rotation of the zygapophyseal joints. It also acts eccentrically to help control lumbar flexion. The primary direction of force is vertical, directly perpendicular to the spinous process to which it attaches. Axial rotation is produced by abdominal oblique muscles, which also produce a flexion force when activated. Thus, the lumbar multifidus is also active in pure axial rotation to provide an extension force that counteracts the tendency of the trunk to flex during rotation. This function has been confirmed by electromyographic studies. The multifidus acts in a polysegmental nature on the vertebrae over which it crosses without inserting, resulting in extension. In this way it increases the lumbar lordosis.
Lumbar Erector Spinae
The lumbar erector spinae are polysegmental muscles consisting of the longissimus and the iliocostalis, whose anatomic configurations differ depending on the spinal location from which they arise. The longissimus fibers are lateral to the multifidus fibers and just medial to the iliocostalis fibers. These muscles are innervated by the dorsal rami in an overlapping, polysegmental nature.
Longissimus
The lumbar portion of the longissimus consists of five fascicles that arise from a medial, dorsal lumbar transverse process. The L1-4 tendons of the lumbar longissimus form the lumbar intermuscular aponeurosis and insert into the medial PSIS. The L5 fascicle inserts just medial to this aponeurosis. Unilateral contraction of the lumbar longissimus results in lateral lumbar flexion. Bilateral contraction results in vertebral motions of extension by way of posterior sagittal rotation and posterior translation. Interestingly, longissimus extension is more pronounced at successively higher levels up the lumbar spine, whereas posterior translation is more pronounced at successively lower levels down the lumbar spine.
The thoracic portion of the longissimus extends from attachments to the thoracic transverse processes and ribs of T1 or T2 to T12, thus consisting of 11 or 12 pairs of fascicles. The tendons descend, forming small muscle bellies then long tendons that join to form the erector spinae aponeurosis, which overlies the lumbar longissimus. The distal insertion points range from the L3 to S3 spinous processes and along a line on the sacrum from the S3 spinous process to the inferior PSIS. The higher up in the thoracic spine the fibers arise, the more medially and superiorly they insert, such that only the fibers from T6 or T7 to T12 actually cross the entire lumbar spine. Its primary action is across the thoracic spine, but it causes indirect lumbar extension, increasing the lumbar lordosis.
Iliocostalis
The lumbar portion of the iliocostalis has attachments from the dorsal, lateral L1 to L4 transverse processes and just lateral to the middle layer of the thoracolumbar fascia. The distal attachments are along the iliac crest just lateral to the PSIS. The higher in the lumbar spine the fibers arise, the more medially they insert. Interestingly, there is an L5 fascicle at birth that becomes replaced by collagen to contribute to the formation of a ligament, the iliolumbar ligament, by the third decade. Similarly to the lumbar portion of the longissimus, unilateral contraction produces lateral lumbar flexion and bilateral contraction produces extension by posterior rotation combined with posterior translation, the latter especially at lower lumbar fibers. With the more lateral attachments, the iliocostalis can produce some axial rotation. Similarly to the multifidus, the iliocostalis can help resist the flexion moment of the abdominal oblique muscles, thus allowing them to produce pure axial rotation.
The thoracic component of the iliocostalis consists of two parts, one that arises from the thoracic spine and inserts into the lumbar spine and another that is contained to the thoracic spine. The former has attachments from the medial, posterior T5 or T6 to T12 ribs, with each fascicle arising from the angle of the rib. Similarly to the thoracic portion of longissimus, this muscle has proximal tendinous attachments that form small muscle bellies that form long caudal tendons. These tendons also contribute to the erector spinae aponeurosis, but their attachments are to the PSIS and iliac crest. The action of these fibers causes a direct extension of the thoracic spine and indirect extension of the lumbar spine, increasing the lumbar lordosis. Unilateral contraction of the muscle also produces direct lateral flexion of the rib cage and indirect lateral flexion of the lumbar spine. Ipsilateral contraction of this muscle may serve to control contralateral axial rotation of the rib cage and lumbar spine.
Erector Spinae Aponeurosis
As described earlier, this layer of joined tendinous fibers is composed of the caudal tendons of the longissimus and iliocostalis fibers that span from the thoracic to the lumbar spine. It also includes small contributions from superficial upper lumber multifidus fibers. The lumbar portions of the longissimus and iliocostalis do not contribute to the aponeurosis and can act independently of it.
Thoracolumbar Fascia
Three layers of fascia envelope the lumbar muscles: anterior, middle, and posterior. The anterior layer is anterior to the quadratus lumborum and the middle layer is posterior to the quadratus lumborum. Both the anterior and middle layers attach medially to the transverse process tips and then blend with the intertransverse ligaments. The posterior layer lies posterior to erector spinae muscles, attaching medially to the lumbar and sacral spinous processes. The posterior layer itself is divided into two layers, or laminae, whose fibers are oriented in a criss-cross pattern. The superficial lamina is continuous with the latissimus dorsi aponeurosis laterally and the deep lamina attaches laterally to the PSIS and posterior iliac crest and blends in with the erector spinae aponeurosis. The anterior, middle, and posterior layers of the thoracolumbar fascia blend laterally to the iliocostalis and form the lateral raphe . This connective tissue line serves as an attachment for the transversus abdominis aponeurosis and the inferior oblique muscle.
Abdominal Muscles
Although extrinsic to the lumbar spine, the rectus abdominis, external oblique, internal oblique, and transversus abdominis are important drivers of lumbar motion and control. The transversus is deepest and, as discussed earlier, attaches indirectly to the lumbar spine via the thoracolumbar fascia with horizontally oriented fibers. It appears to function as a built-in corset of the spine, with activity varying in relation to postural demands. It can be activated in isolation by bringing the umbilicus posteriorly toward the spine or “hollowing” the abdomen. Just superficial to transversus is the internal oblique muscle, with the external oblique superficial to that. The oblique abdominal muscles, rather than lumbar back muscles, are primarily responsible for axial control during rotation movements. Finally, the rectus abdominis spans from the inferior chest wall to the pubis and is a lumbar/trunk flexor.
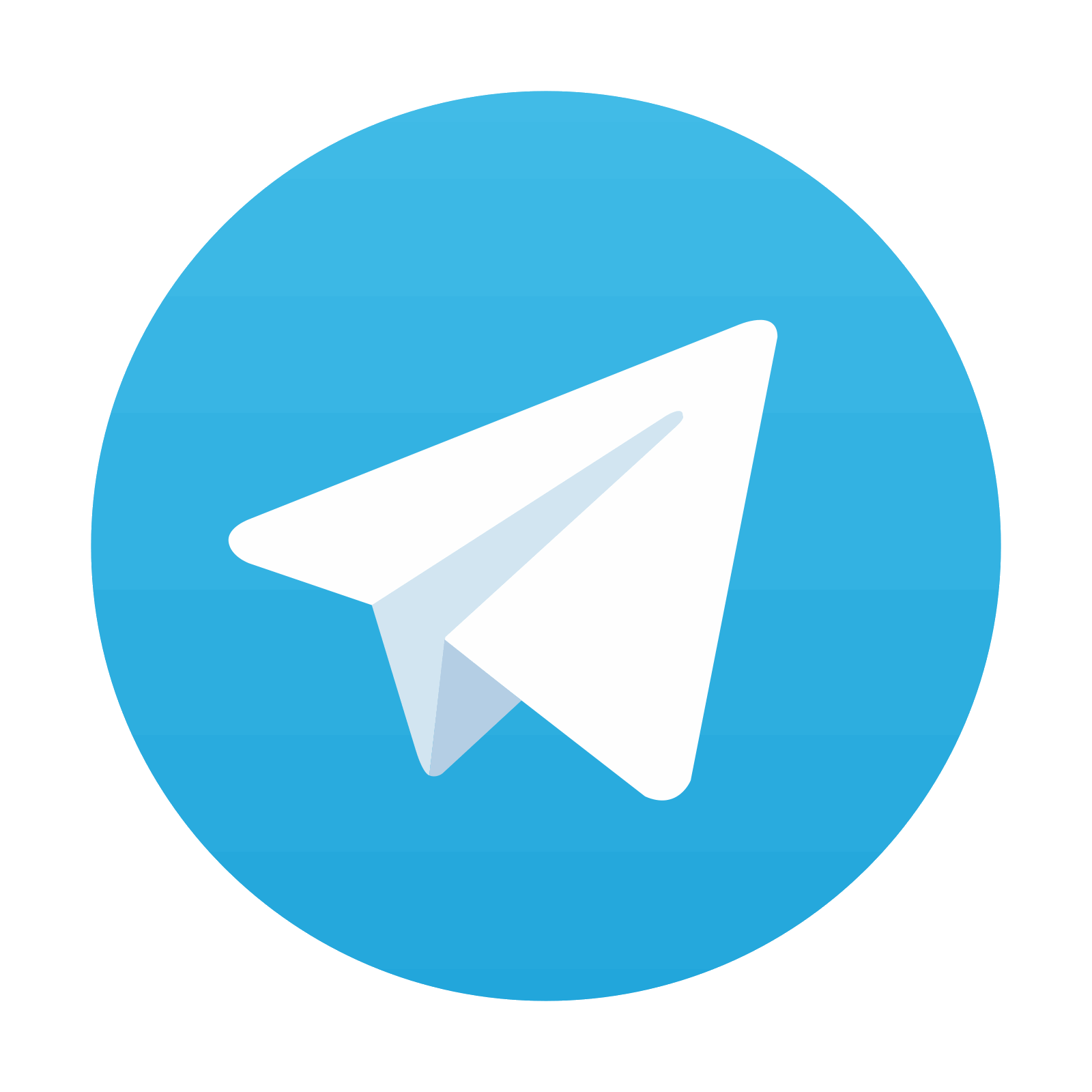
Stay updated, free articles. Join our Telegram channel
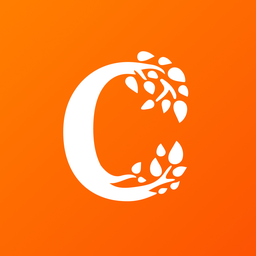
Full access? Get Clinical Tree
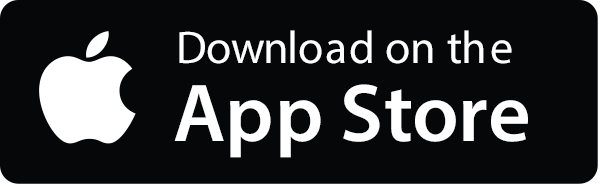
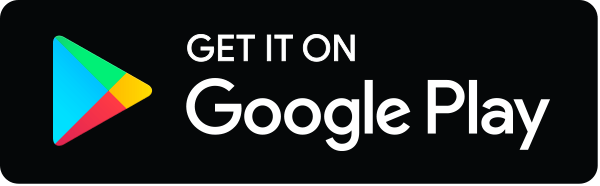