Glioblastoma remains one of the most difficult cancers to treat and represents the most common primary malignancy of the brain. Although conventional treatments have found modest success in reducing the initial tumor burden, infiltrating cancer cells beyond the main mass are responsible for tumor recurrence and ultimate patient demise. Targeting residual infiltrating cancer cells requires the development of new treatment strategies. The emerging field of cancer nanotechnology holds promise in the use of multifunctional nanoparticles for imaging and targeted therapy of glioblastoma. This article examines the current state of nanotechnology in the treatment of glioblastoma and directions of further study.
- •
GBM remains a difficult tumor to treat due to its infiltrative nature.
- •
Nanoparticles present a new way to target infiltrating cells.
- •
Magnetic nanoparticles (MNPs) can be used as MRI contrast agents as well as therapeutic agents by the use of thermotherapy.
- •
In a nanoparticle formulation chemotherapeutics can be more efficacious than conventional chemotherapeutic agents due to their ability to target GBM cells and release drug.
- •
Gene delivery through the use of nanoparticles may be a safe option to deliver therapeutic genes to tumor cells.
- •
Brachytherapy delivered by radioactive nanoparticles can provide long-term focused radiation therapy to these lesions.
- •
Gold nanoparticles can be used to treat tumors through phototherapy, where deep penetrating near-infrared light can be used to inhibit tumor growth.
- •
Nanoparticles can be delivered safely systemically or by bulk flow using convection-enhanced delivery (CED) directly to the tumor.
- •
Magnetic targeting can be used to enhance the delivery of MNPs by directing the delivered particles to the area of interest.
Introduction
Glioblastoma (GBM) is the most common primary malignancy of the brain as well as its most malignant. The median survival after radiation and chemotherapy ranges from 12 to 15 months, despite advances in surgery, radiation, and chemotherapy. GBM tumors are nearly uniformly fatal due to local recurrence. Even for lesions amenable to gross surgical resection, infiltrating cancer cells beyond the boundaries of the enhancing lesion are responsible for tumor recurrence as well as radiation and chemotherapy resistance.
Cancer nanotechnology has recently emerged as a field that may provide answers to some of the difficulties encountered in treating GBM. Nanoparticles, defined as particles less than 100 nm in hydrodynamic size, have been used in the treatment of various cancers. The use of biocompatible nanomaterials has permitted the fabrication of nanoparticles with capabilities that surpass those of conventional agents. Chemotherapy-loaded nanoparticles have resulted in sustained-release formulations that can lower systemic toxicity and produce greater antitumor effects. Recently developed nanoparticles can cross the blood-brain barrier (BBB) after systemic administration or be distributed in the brain by CED to target GBM cells therapeutically while harboring elements that may enable imaging of the particle and the target. The field has been moving at a rapid pace, enabling nanoparticles to be used in recent clinical trials. Although not exhaustive, the list of nanoparticles used in the treatment of experimental GBM includes polymeric particles, micelles, nanoshells, quantum dots, and magnetic iron oxide nanoparticles (IONPs). Nanotubes are another formulation of nanoparticle, used to create structures that can trap diagnostic or therapeutic modalities within a cage. This article discusses the use of different nanoparticle formulations in strategies to image and treat GBM, including delivery schemes.
Magnetic nanoparticles
MRI Contrast Properties of MNPs
The base of the promise for theranostic nanoparticles with both therapeutic and diagnostic ability hinges on the idea that such nanoparticles will be able to image where the lesion is and treat it. MNPs have attracted particular interest in this respect due to their unique paramagnetic properties that enable their detection by MRI. These MNPs have shown great potential as T 1 or T 2 contrast agents in MRI, with superparamagnetic iron oxide–based nanoparticles (SPIOs) as the most commonly investigated type of MRI contrast agents. Since 1990, ultrasmall SPIOs (USPIOs), smaller than 50 nm, have been considered an MRI contrast agent, and most of the MRI data regarding nanoparticles references these particles. USPIOs can be visualized in T 2 -weighted MRI sequences (T 2 contrast agents) as a hypointense (dark) signal (negative contrast enhancement) or with T 1 -weighted MRI sequences (T 1 contrast agents) as a hyperintense (bright) signal (positive contrast enhancement).
USPIOs can provide contrast for a longer period of time compared with gadolinium (Gd)-based contrast agents that are rapidly eliminated by the kidney. USPIOs are also taken up by tumor cells as well as by reactive phagocytic cells (eg, microglia) found in brain tumors. The USPIOs can reside within brain tumors much longer than Gd-based agents, with a peak enhancement noted at 24 to 28 hours and persisting up to 72 hours after administration. These agents may provide a safe alternative for patients at risk for nephrogenic systemic fibrosis, because preliminary studies have shown no adverse renal effects.
MNPs for Targeted Brain Tumor Imaging
Targeting of tumor cells can increase the benefits provided by nanoparticles as contrast agents. IONPs are taken up by GBM cells both in vivo and in vitro. Surface functionalization further enhances tumor uptake of these particles. Tumor-specific ligands conjugated to MNPs can further enhance the uptake within targeted tumor tissue ( Fig. 1 ). Antibodies, peptides (including toxins), cytokines, and chemotherapeutic agents have been reported as possible MNP ligands. Amphiphilic triblock copolymer IONPs can be conjugated with a purified antibody that selectively binds to the epidermal growth factor receptor (EGFR) deletion mutant, EGFR vIII, which is solely expressed by a population of GBM tumors. Such nanoparticles exhibit MR contrast enhancement of GBM cells and can target these therapy-resistant cancer cells in vitro and in vivo.

Chlorotoxin, derived from scorpion venom, specifically binds to matrix metalloproteinase 2 (MMP-2), which is overexpressed on the surface of GBM cells. MMP-2 degrades the extracellular matrix during tumor invasion, and chlorotoxin can be used to bind the MMP-2 and inhibit infiltration. Chlorotoxin conjugated to MNPs can act as MRI contrast agents and the addition of a Cy5.5 molecule makes these suitable for use as an intraoperative fluorescent dye as well.
F3 is a small peptide that specifically binds to nucleolin overexpressed on proliferating endothelial cells of tumor cells and the associated vasculature. F3-coated IONPs can provide significant MRI contrast enhancement of intracranial rat-implanted tumors, compared with noncoated F3 nanoparticles, when administered intravenously.
A molecular MRI contrast agent, consisting of SPIO coated with dextran, was functionalized with an anti–insulinlike growth factor binding protein 7 (anti-IGFBP7) single-domain antibody and was found by both MRI and in vivo fluorescent imaging to target the vasculature of GBM cells.
Gd has also been incorporated into some therapeutic nanoparticles to enable them to be tracked using MRI. One group has designed nanoparticles containing Gd, which are rapidly taken up by the GL261 tumor cell line and show MRI contrast when these cells are then cultured in a chick embryo host. Gd nanoparticles functionalized with diethylenetriaminepentaacetic acid (DTPA) can also be used as a radiosensitizing agent. Fullerene magnetic nanotubes have been made such that Gd can be trapped within these structures to make them an effective contrast agent, along with whatever therapeutic modality is also associated with the fullerene cage. It is also possible to internalize IONPs in these larger nanotube structures so that the magnetic properties of iron oxide can be used, allowing clinicians to localize these particles to a particular area. This, together with surface targeting, can greatly increase the amount of intake and resultant therapeutic effect of these particles.
MNPs for Optical Delineation of Brain Tumors
Although surgical intervention is not curative in GBM, obtaining a maximal resection is important for survival. The use of intraoperative MRI and neuronavigation has increased extent of resection and outcome. Recently, fluorescence-guided surgery after oral administration of 5-aminolevulinic acid (5-ALA) has resulted in more complete resection of malignant gliomas. Laboratory studies have attempted to find ways to use optical aides to increase the contrast between normal and tumor tissue, and these methods have shown improvement in the extent of tumor resection in clinical use.
Fluorescent molecules have already been successfully incorporated into several nanoparticles. An IONP-Cy5.5 molecule has been used in many preclinical studies, giving it the dual benefits of MRI detection and possibly enhanced surgical contrast using the fluorescent properties of the particle. This also could lead to theranostic particles that could be injected preoperatively to outline malignant tissue that would need to be resected at surgery.
MNPs for Stem Cell Tracking
The ability of MNPs to act as MRI contrast agents can be used to track stem cell tropism to malignant brain tumors in vivo. Intracranially administered neural stem cells have tropism for GBM tumors, making them attractive for tumor-targeting gene therapy. Mesenchymal stem cells have also been found to migrate to tumor cells. By labeling these cells with IONPs, this migration can be visualized on MRI. Magnetically labeled hematopoietic stem cells can also be tracked to gliomas in this fashion.
MNPs for Thermotherapy of GBM
One of the more unique features of MNPs is the ability to induce hyperthermia when exposed to alternating magnetic fields. Temperature elevations in the range of 41°C to 46°C can cause cells to undergo heat stress, resulting in protein denaturation, protein folding, aggregation, and DNA cross-linking. This process can induce apoptosis and heat shock protein expression. At the tissue level, moderate hyperthermia causes changes in pH, perfusion, and oxygenation of the tumor microenvironment. These effects, combined with chemotherapy and radiation, can have a synergistic effect.
Hyperthermia can be induced in MNPs through the use of an appropriate alternating magnetic field of the right amplitude and frequency to heat up the nanoparticles. A predictable and sufficient amount of heat known as the specific absorption rate is produced. The MNPs use several different mechanisms to convert the magnetic energy into heat energy. Néel relaxation is caused by rapidly occurring changes in the direction of magnetic moments relative to crystal lattice. Brownian relaxation results from the physical rotation of MNPs within the medium in which they are placed. Both internal (Néel) and external (brownian) sources of friction lead to a phase lag between applied magnetic field and the direction of magnetic moment, producing thermal losses ( Fig. 2 ).

MNPs can be specifically engineered to maximize their suitability for hyperthermia by producing greater saturation magnetization, optimal anisotropy, and larger size within the constraints of nanoparticle production. MNPs suitable for thermotherapy can be made from a combination of various metals, including manganese (Mn), iron (Fe), cobalt (Co), nickel (Ni), zinc (Zn), and magnesium (Mg) and their oxides. Ferrites of the various metals are frequently used in these settings, such as cobalt ferrites (CoFe 2 O 4 ), manganese ferrites (MnFe 2 O 4 ), nickel ferrites (NiFe 2 O 4 ), lithium ferrites (Li 0.5 Fe 2.5 O 4 ), mixed ferrites of nickel–zinc–copper, and cobalt–nickel ferrites. There are also ferromagnetic nanoparticles that are iron based and have greater magnetic properties than IONPs. These cobalt ferrites–based nanoparticles produce greater hyperthermia effects at much lower concentrations than IONPs. FeNPs are comprised of an iron core surrounded by an iron oxide layer to permit stability. Nevertheless, owing to their lack of toxicity, excellent biocompatibility, and their capacity to be metabolized, iron oxide–based MNPs are actively being studied for thermotherapy of brain tumors.
MNP-based hyperthermia has been evaluated for feasibility in animal models and in human patients with malignant brain tumors. Dextran-coated or aminosilane-coated IONPs have been used for thermotherapy in a rodent GBM model and in a human clinical trial in patients with recurrent GBM. Intratumoral injection of aminosilane-coated IONPs (core size 12 nm) and application of an alternating magnetic field (100 kHz) in several sessions before and after adjuvant fractionated radiation therapy was given. With a high concentration of IONPs (>100 mg/mL), this achieved effective thermotherapy with a median peak temperature within the tumor of 51.2°C. This phase II clinical trial successfully demonstrated safety and efficacy of thermotherapy of malignant brain tumors with MNPs in humans, with a significant increase in overall survival compared with a reference population. Further randomized studies will be required to validate the promise of this treatment modality.
Magnetic nanoparticles
MRI Contrast Properties of MNPs
The base of the promise for theranostic nanoparticles with both therapeutic and diagnostic ability hinges on the idea that such nanoparticles will be able to image where the lesion is and treat it. MNPs have attracted particular interest in this respect due to their unique paramagnetic properties that enable their detection by MRI. These MNPs have shown great potential as T 1 or T 2 contrast agents in MRI, with superparamagnetic iron oxide–based nanoparticles (SPIOs) as the most commonly investigated type of MRI contrast agents. Since 1990, ultrasmall SPIOs (USPIOs), smaller than 50 nm, have been considered an MRI contrast agent, and most of the MRI data regarding nanoparticles references these particles. USPIOs can be visualized in T 2 -weighted MRI sequences (T 2 contrast agents) as a hypointense (dark) signal (negative contrast enhancement) or with T 1 -weighted MRI sequences (T 1 contrast agents) as a hyperintense (bright) signal (positive contrast enhancement).
USPIOs can provide contrast for a longer period of time compared with gadolinium (Gd)-based contrast agents that are rapidly eliminated by the kidney. USPIOs are also taken up by tumor cells as well as by reactive phagocytic cells (eg, microglia) found in brain tumors. The USPIOs can reside within brain tumors much longer than Gd-based agents, with a peak enhancement noted at 24 to 28 hours and persisting up to 72 hours after administration. These agents may provide a safe alternative for patients at risk for nephrogenic systemic fibrosis, because preliminary studies have shown no adverse renal effects.
MNPs for Targeted Brain Tumor Imaging
Targeting of tumor cells can increase the benefits provided by nanoparticles as contrast agents. IONPs are taken up by GBM cells both in vivo and in vitro. Surface functionalization further enhances tumor uptake of these particles. Tumor-specific ligands conjugated to MNPs can further enhance the uptake within targeted tumor tissue ( Fig. 1 ). Antibodies, peptides (including toxins), cytokines, and chemotherapeutic agents have been reported as possible MNP ligands. Amphiphilic triblock copolymer IONPs can be conjugated with a purified antibody that selectively binds to the epidermal growth factor receptor (EGFR) deletion mutant, EGFR vIII, which is solely expressed by a population of GBM tumors. Such nanoparticles exhibit MR contrast enhancement of GBM cells and can target these therapy-resistant cancer cells in vitro and in vivo.
Chlorotoxin, derived from scorpion venom, specifically binds to matrix metalloproteinase 2 (MMP-2), which is overexpressed on the surface of GBM cells. MMP-2 degrades the extracellular matrix during tumor invasion, and chlorotoxin can be used to bind the MMP-2 and inhibit infiltration. Chlorotoxin conjugated to MNPs can act as MRI contrast agents and the addition of a Cy5.5 molecule makes these suitable for use as an intraoperative fluorescent dye as well.
F3 is a small peptide that specifically binds to nucleolin overexpressed on proliferating endothelial cells of tumor cells and the associated vasculature. F3-coated IONPs can provide significant MRI contrast enhancement of intracranial rat-implanted tumors, compared with noncoated F3 nanoparticles, when administered intravenously.
A molecular MRI contrast agent, consisting of SPIO coated with dextran, was functionalized with an anti–insulinlike growth factor binding protein 7 (anti-IGFBP7) single-domain antibody and was found by both MRI and in vivo fluorescent imaging to target the vasculature of GBM cells.
Gd has also been incorporated into some therapeutic nanoparticles to enable them to be tracked using MRI. One group has designed nanoparticles containing Gd, which are rapidly taken up by the GL261 tumor cell line and show MRI contrast when these cells are then cultured in a chick embryo host. Gd nanoparticles functionalized with diethylenetriaminepentaacetic acid (DTPA) can also be used as a radiosensitizing agent. Fullerene magnetic nanotubes have been made such that Gd can be trapped within these structures to make them an effective contrast agent, along with whatever therapeutic modality is also associated with the fullerene cage. It is also possible to internalize IONPs in these larger nanotube structures so that the magnetic properties of iron oxide can be used, allowing clinicians to localize these particles to a particular area. This, together with surface targeting, can greatly increase the amount of intake and resultant therapeutic effect of these particles.
MNPs for Optical Delineation of Brain Tumors
Although surgical intervention is not curative in GBM, obtaining a maximal resection is important for survival. The use of intraoperative MRI and neuronavigation has increased extent of resection and outcome. Recently, fluorescence-guided surgery after oral administration of 5-aminolevulinic acid (5-ALA) has resulted in more complete resection of malignant gliomas. Laboratory studies have attempted to find ways to use optical aides to increase the contrast between normal and tumor tissue, and these methods have shown improvement in the extent of tumor resection in clinical use.
Fluorescent molecules have already been successfully incorporated into several nanoparticles. An IONP-Cy5.5 molecule has been used in many preclinical studies, giving it the dual benefits of MRI detection and possibly enhanced surgical contrast using the fluorescent properties of the particle. This also could lead to theranostic particles that could be injected preoperatively to outline malignant tissue that would need to be resected at surgery.
MNPs for Stem Cell Tracking
The ability of MNPs to act as MRI contrast agents can be used to track stem cell tropism to malignant brain tumors in vivo. Intracranially administered neural stem cells have tropism for GBM tumors, making them attractive for tumor-targeting gene therapy. Mesenchymal stem cells have also been found to migrate to tumor cells. By labeling these cells with IONPs, this migration can be visualized on MRI. Magnetically labeled hematopoietic stem cells can also be tracked to gliomas in this fashion.
MNPs for Thermotherapy of GBM
One of the more unique features of MNPs is the ability to induce hyperthermia when exposed to alternating magnetic fields. Temperature elevations in the range of 41°C to 46°C can cause cells to undergo heat stress, resulting in protein denaturation, protein folding, aggregation, and DNA cross-linking. This process can induce apoptosis and heat shock protein expression. At the tissue level, moderate hyperthermia causes changes in pH, perfusion, and oxygenation of the tumor microenvironment. These effects, combined with chemotherapy and radiation, can have a synergistic effect.
Hyperthermia can be induced in MNPs through the use of an appropriate alternating magnetic field of the right amplitude and frequency to heat up the nanoparticles. A predictable and sufficient amount of heat known as the specific absorption rate is produced. The MNPs use several different mechanisms to convert the magnetic energy into heat energy. Néel relaxation is caused by rapidly occurring changes in the direction of magnetic moments relative to crystal lattice. Brownian relaxation results from the physical rotation of MNPs within the medium in which they are placed. Both internal (Néel) and external (brownian) sources of friction lead to a phase lag between applied magnetic field and the direction of magnetic moment, producing thermal losses ( Fig. 2 ).
MNPs can be specifically engineered to maximize their suitability for hyperthermia by producing greater saturation magnetization, optimal anisotropy, and larger size within the constraints of nanoparticle production. MNPs suitable for thermotherapy can be made from a combination of various metals, including manganese (Mn), iron (Fe), cobalt (Co), nickel (Ni), zinc (Zn), and magnesium (Mg) and their oxides. Ferrites of the various metals are frequently used in these settings, such as cobalt ferrites (CoFe 2 O 4 ), manganese ferrites (MnFe 2 O 4 ), nickel ferrites (NiFe 2 O 4 ), lithium ferrites (Li 0.5 Fe 2.5 O 4 ), mixed ferrites of nickel–zinc–copper, and cobalt–nickel ferrites. There are also ferromagnetic nanoparticles that are iron based and have greater magnetic properties than IONPs. These cobalt ferrites–based nanoparticles produce greater hyperthermia effects at much lower concentrations than IONPs. FeNPs are comprised of an iron core surrounded by an iron oxide layer to permit stability. Nevertheless, owing to their lack of toxicity, excellent biocompatibility, and their capacity to be metabolized, iron oxide–based MNPs are actively being studied for thermotherapy of brain tumors.
MNP-based hyperthermia has been evaluated for feasibility in animal models and in human patients with malignant brain tumors. Dextran-coated or aminosilane-coated IONPs have been used for thermotherapy in a rodent GBM model and in a human clinical trial in patients with recurrent GBM. Intratumoral injection of aminosilane-coated IONPs (core size 12 nm) and application of an alternating magnetic field (100 kHz) in several sessions before and after adjuvant fractionated radiation therapy was given. With a high concentration of IONPs (>100 mg/mL), this achieved effective thermotherapy with a median peak temperature within the tumor of 51.2°C. This phase II clinical trial successfully demonstrated safety and efficacy of thermotherapy of malignant brain tumors with MNPs in humans, with a significant increase in overall survival compared with a reference population. Further randomized studies will be required to validate the promise of this treatment modality.
Nanoparticalized chemotherapeutic agents
Although few conventional chemotherapeutics have been proved effective in GBM, chemotherapeutics in a nanoparticle formulation offer possible advantages. These often can be targeted, evade the reticuloendothelial system for prolonged circulatory time, and potentially cross the BBB better then standard chemotherapy agents. Polyethylene glycol (PEG)-coated paclitaxel (taxol) nanoparticles have been shown to offer superior bioavailability compared with free paclitaxel with a survival advantage shown in a rodent glioma model. Poly(d,l-lactide-co-glycolide) (PLGA) nanoparticles are another form of biocompatible nanoparticles. CED of these nanoparticles, loaded with camptothecin, has been shown efficacious in a rodent glioma model. Although the controlled release offered by nanoparticles can reduce systemic toxicity and allow drug to be slowly released only when it has reached its target, there is also a need to ensure that an adequate dose is delivered to the lesion being treated. Nanoparticles have been developed that are thermosensitive, releasing their drug preferentially when the temperature has been increased. When delivered with gold nanorods, concurrent photothermal hyperthermia can release the drug from the heat sensitive nanoparticle, thus increasing efficacy.
Gene delivery with nanoparticles
The Cancer Genome Atlas has revealed the multiple genetic aberrations in GBM tumors that can serve as therapeutic targets provide targets. Cationic solid lipid nanoparticles can be conjugated to PEGylated therapeutic c-Met small interfering RNA and reduce human GBM tumor growth in a rodent model without significant toxicity. Another nanoparticle, containing the integrin-binding motif RGD, together with the PEG-polyethylenimine (PEI) nonviral gene carrying nanoparticle, was able to deliver a plasmid expressing the tumor necrosis factor-related apopotosis-inducing ligand with increased efficiency and increase survival in a rodent glioma model.
Nanoparticles for brachytherapy
Brachytherapy, where localized radiotherapy is delivered directly to a tumor, has been explored as a strategy with nanoparticles. In an orthotopic xenograft brain tumor model, a functionalized fullerene nanoparticle ( 177 Lu-DOTA-f-Gd 3 N@C 80 ), with radiolabeled lutetium 177 ( 177 Lu) and tetraazacyclododecane tetraacetic acid (DOTA), provided an anchor to deliver effective brachytherapy and longitudinal imaging of the tumor. Internal fractionated radiation has also been achieved using a lipid nanoparticle formulation of radionucliides, such as 188 Re-SSS in the 9L rat glioma cell line.
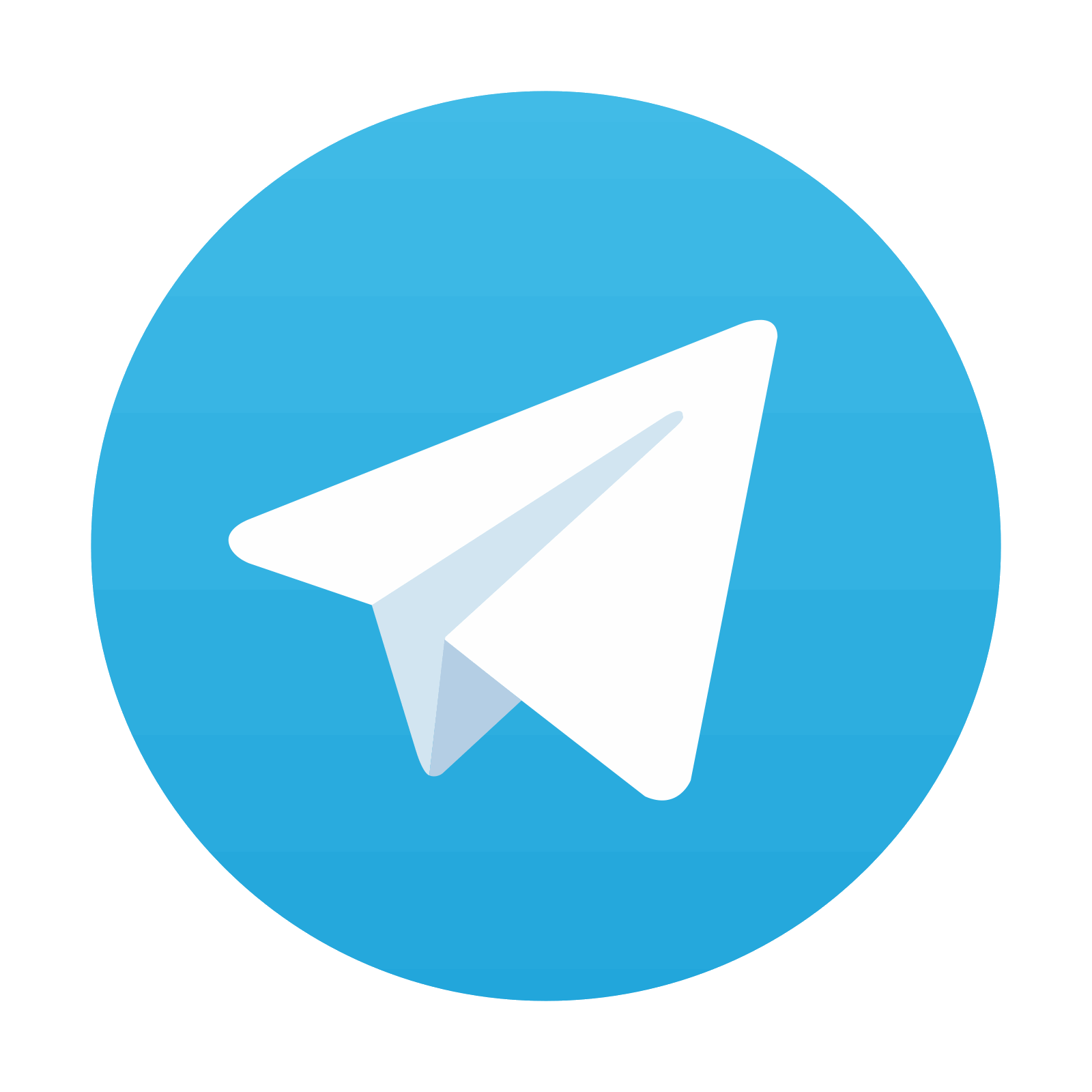
Stay updated, free articles. Join our Telegram channel
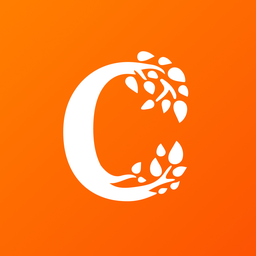
Full access? Get Clinical Tree
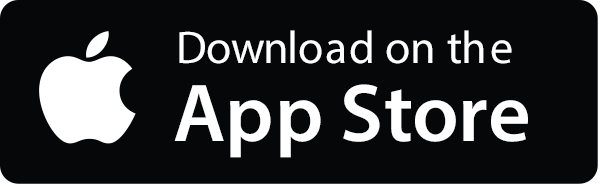
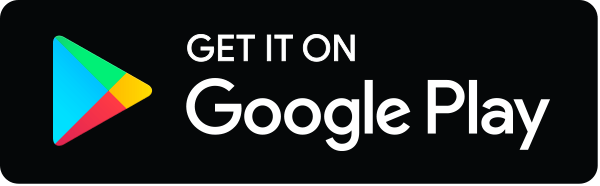