Epileptic seizures are stereotypical patterns of abnormal synchronized activity among different cellular populations. They are products of pathological activation of networks involved in controlling the temporal (initiation, maintenance, termination) or semiological evolution of these ictal events. Often seizure activity forms a reverberating circuit that can perpetuate and self-sustain, as is the case with temporal lobe seizures. Alternatively, a seizure can be paroxysmal but temporally confined, albeit with the potential to recur, such as in epileptic myoclonus or spasms. The basic elements of these networks may belong to a single or multiple systems, which are recognized based on well-identified physiological functions. As such, they are useful in localizing seizure activity and defining seizure propagation. Communications within a system or between different systems, during a seizure, can be via anatomical connections (i.e., afferent or efferent neuronal projections) or humoral (i.e., hormonal, metabolic or immune responses). A sine qua non of epilepsy is the propensity for unprovoked seizures to recur. In certain cases, this is already a predetermined feature of the original epileptic seizure network, as occurs in certain types of primary idiopathic epilepsies, that is, absence. The expression of the seizures, in such cases, appears to depend more on modifiers involved in the physiological maturation of the network, such as age or hormonal factors. In other types of epilepsy, the epileptic predisposition is not as strong initially, but dynamic changes of the network triggered by seizures or unrelated epigenetic factors promote epileptogenesis. The classical example is temporal lobe epilepsy (TLE), whereby initial precipitating events may increase the likelihood that TLE will manifest.
Epileptic networks include an initiating circuit, which, in certain cases, may be ignited by endogenous or exogenous triggers (such as stress, fever, photic stimulation, and sleep–wake cycle). Seizure activity may remain regionally contained or spread to secondary domains, via propagation pathways. Initiating networks are different for generalized or localization-related epilepsies. Identification of the networks and systems involved in seizures and epilepsy is important for their correct localization, classification, and treatment, as well as the identification of methods to predict their recurrence and their outcome. In this review, we will summarize some of the known networks and systems implicated in common types of epileptic seizures and syndromes, discuss them in the context of specific types of epileptic seizures, and review factors that can modify them.
Probably, the most popular focus of attention in epilepsy research has been the mesial temporal lobe structures, due to their high epileptogenicity and the high incidence of TLE. Medial temporal lobe structures are the dentate gyrus (DG), hippocampus, subiculum, pre- and parasubiculum, entorhinal, perirhinal, and parahippocampal cortices. These are normally involved in long-term memory formation, consolidating information obtained through connections with associational cortices. The basic circuit interconnects the subiculum, DG, and cornu ammonis regions of the hippocampus (CA3 and CA1) (a.k.a. trisynaptic pathway), with the entorhinal (EC) and amygdalar cortices (Fig. 2–1).1 The major excitatory input to the DG originates at layer II of the EC and enters into the ipsilateral hippocampus through the perforant pathway. Granule neurons of the DG project onto area CA3 via the mossy fiber pathway. CA3 pyramidal neurons interact with CA1 pyramidal neurons through the Schaffer collaterals. Finally, CA1 neurons send axons to the subiculum, which in turn projects to cortical areas. The web of connections among different areas is actually more complex, as individual areas can project to contralateral hippocampus (i.e., CA3 neurons or hilar neurons) or have antidromic influences within the hippocampus (i.e., CA3 toward hilus or DG; CA1 toward CA3) or between the hippocampus and cortical areas (i.e., temporoammonic pathway connecting CA1–CA3 neurons to layer III of EC). Furthermore, local interneurons, classically operating via inhibitory signaling systems, such as gamma-aminobutyric acid (GABA), may modify the activity of this circuit. The medial temporal structures are also connected with the medial thalamus and inferior frontal lobes.2 Midline thalamic nuclei act as synchronizers of the neuronal activity that reverberates through this network. Escape of seizure activity toward the cortex, directly or through the thalamus, or toward the brainstem allows seizure generalization and can impair consciousness.3
Figure 2–1.
Schematic representation of hippocampal connections. 1, perforant pathway; 2, mossy fiber pathway; 3, Schaffer collaterals; 4, temporoammonic pathway; MEA, medial entorhinal cortex; LEA, lateral entorhinal cortex. (Based on information from Amaral D, Lavenex P. Hippocampal Neuroanatomy. New York: Oxford University Press, 2007.)

This network can be further modulated by subcortical influences. The septohippocampal pathway originates in the medial septal nucleus and diagonal band of Broca and enters the hippocampus through the fimbria/fornix, operating via cholinergic and GABAergic mechanisms. The cholinergic fibers interact with most hippocampal regions, whereas GABAergic ones modulate the basket cells of DG.1 Cholinergic afferents from brainstem nuclei (pedunculopontine nucleus and pontomesencephalic reticular formation) participate in rapid eye movement (REM) sleep regulation. The septohippocampal is an important relay pathway, given its connections with the amygdala, hypothalamus, and brainstem, and plays critical role in the generation of the theta rhythm, which is important for learning and memory.4 The brainstem monoamine pathways provide noradrenergic control from the locus coeruleus, targeting particularly CA3 and dentate hilar neurons, serotoninergic input from the raphe nuclei, and dopaminergic input from the ventral tegmental area. Noradrenergic input to the hippocampus enhances excitability and promotes long-term potentiation. Serotoninergic and dopaminergic pathways are primarily inhibitory and participate in sleep–wake transitions.1
In addition to the temporal lobe, functional neuroimaging, clinical, electrographic, and anatomic data have indicated the existence of additional distinct networks involving other cortical regions2: medial occipital–lateral temporal, superior parietal–medial frontal, bifrontal–pontine–subthalamic, and parietal–medial temporal.
The thalamus has central role in rhythm generation and sensory information trafficking in the brain.5 First, it can act as a relay system forwarding information from multiple subcortical centers to the cerebral cortex. In seizures, this can lead to seizure spreading. Second, if the level of afferent information decreases, thalamus resumes its role as a generator of oscillatory rhythms observed in sleep, such as sleep spindles, by inducing neocortical cells to fire bursts.6,7 These corticothalamic networks are important, as they can drive the expression of spike-wave (SW) discharges. Third, midline intralaminar thalamic nuclei project diffusely to the cortex, allowing diffuse activation of cortical areas. Utilization of this gateway may allow for seizure generalization. The principal sensory relay nuclei of the thalamus receive excitatory glutamatergic afferents from the cortex and send excitatory glutamatergic projections to the same cortical areas. Thalamic and cortical neurons both project to and excite the nucleus reticularis thalami (NRT) GABAergic interneurons, which in turn project onto and inhibit the thalamic relay neurons (GABAergic projections). Midline intralaminar thalamic nuclei project to the frontal, medial, and dorsolateral cortex in a more diffuse manner compared with relay neurons, as well as to the striatum; they receive afferents from cortex, globus pallidus, and NRT interneurons. In addition, thalamus receives afferents from brainstem structures (locus coeruleus, raphe nuclei, pedunculopontine, and lateral tegmental nuclei) and the basal forebrain and hypothalamus.5
During wakefulness, corticothalamic neurons fire continuously. When sensory input is diminished, thalamic neurons hyperpolarize, leading to deinactivation of low threshold calcium channels (T-channels), and this switches their mode of activity to a synchronized oscillatory pattern (spindles). These oscillations consist of sequential cycles of coactivation of cortical and thalamic relay neurons followed by NRT activation, which then inhibits thalamic neurons and leads to a new cycle of oscillation.8 Bazhenov et al9 have also proposed that sleep-induced NRT hyperpolarization may reverse GABAAergic inhibitory postsynaptic potentials (IPSPs) and this in turn can trigger a low threshold spike and sleep oscillations.
The basal ganglia consist of the striatum (caudate and putamen), globus pallidus externa (GPe) and interna (GPi) (or entopeduncular nucleus), subthalamic nucleus (STN), and substantia nigra. They are extensively interconnected (Fig. 2–2) among themselves as well as with the rest of the cortical and subcortical neurons. As a result, they are involved in motor control, sleep, eye movement, cognitive functions, and affective disorders. Because of their central role in information trafficking and processing, as well as their synaptic organization in the form of loops, they play important role in seizure control. Among the first structures to be studied, in their role in seizure propagation, were the substantia nigra and lenticular nucleus (putamen, globus pallidus). A simplified form of the web of connections depicted in Fig. 2–2, as they pertain to seizure control, includes an input of cortical afferents (striatum), which either directly or indirectly (through the GPe and STN) reaches the output structures [GPi and substantia nigra pars reticulata (SNR)]. These in turn relay the information either to the striatum or the thalamus, superior colliculus, pedunculopontine nuclei, or other brainstem nuclei.
Figure 2–2.
Network of basal ganglia connections. Supplementary Motor, supplementary motor cortex; STN, subthalamic nucleus; GPe, globus pallidus externa; GPi, globus pallidus interna; SNC, substantia nigra pars compacta; SNR, substantia nigra pars reticulata; PPN, pedunculopontine tegmental nucleus. Thalamic nuclei: VA, ventral anterior; CM, centromedian; VL, ventrolateral; Hb, habenular; MD, mediodorsal. Neurotransmitters: Ach, acetylcholine; Glu, glutamate; DA, dopamine; Misc, miscellanea.

Apart from acting as secondary propagation pathways, experimental studies have suggested that certain of these nuclei have the ability to generate seizure-like behaviors. Electrical stimulation or excitation of the inferior colliculus or periaqueductal gray matter elicits running and bouncing clonic seizures; similar manipulations of the reticular system can generate extension or flexion spasms or tonic seizures.10,11,12,13,14 Midbrain tegmental lesions increase the threshold for or prevent hindlimb tonic extension during maximal electroshock seizures (MES), but have no effect on electroshock, flurothyl, or pentylenetetrazole seizure thresholds.15 In these studies (rats), damage to the superior cerebellar peduncle and/or reticular formation was responsible for inhibition of hindlimb extension.13 Other supportive studies have shown that total cerebellectomy abolishes the hindlimb extension component of these seizures. Seizures have also been generated by penicillin injections in the midbrain tegmentum (myoclonic, tonic, and tonic–clonic).16 However, the main focus of interest has been on exploiting the properties of this network to control seizure propagation, as will be discussed later on. Other popular brainstem structures involved in seizure networks are the raphe nuclei and the locus coeruleus. The raphe nuclei consist of serotoninergic neurons that are involved in the control of pain, sleep, and emotions. The noradrenergic projections of locus coeruleus are involved in physiologic responses to stress and panic, REM sleep, autonomic functions, gaze control, and in most seizure models this pathway exerts anticonvulsant effects.17
The best studied type of epilepsy is the mesial TLE (MTLE), which has been linked to characteristic histopathologic alterations in the mesial temporal lobe structures, often referred to as mesial temporal or hippocampal sclerosis (MTS). This is the preponderant histopathological abnormality in patients with intractable TLE, characterized by neuronal loss at specific hippocampal areas (hilar region, CA1, and CA3 pyramidal regions), gliosis, structural and functional reorganization of the hippocampus, including mossy fibers and granular DG cells, and the presence of dysplastic neurons. Although more widespread changes have been shown to occur outside the hippocampus, MTS has concentrated the greatest investigational interest, due to its linkage with intractable epilepsies, the excellent seizure outcomes following temporal lobectomies, and experimental studies showing that prolonged seizures can induce mesial temporal lobe changes, including MTS, in animals that do progress to epilepsy. The initiating circuit in MTLE is thought to include the hippocampus, EC, and midline thalamic nuclei, and reverberates spreading to adjacent structures, such as the amygdala or the temporal neocortex (Fig. 2–3).3 In neocortical TLE, the epileptogenic focus is thought to lie within temporal neocortex but may also spread to the mesial temporal lobe structures. It has been recently reported that the spread of amygdala-kindled seizures occurs first at the SNR, before it reaches the hippocampus or the STN, probably via direct propagation through the amygdalonigral pathway.18 The extensive connectivity of the hippocampus and temporal lobe with other brain structures (Figs. 2–1 and 2–3) explains the diversity in semiology or associated symptoms observed in TLE patients. The sleep-induced aggravation of interictal and ictal events can be mediated by the brainstem connections with centers involved in sleep–wake cycle. Certain ictal signs have also been localized to different areas of the network, including fear or olfactory auras (amygdala, hippocampus, and parahippocampal areas), experiential auras or early unilateral somatic motor involvement without automatisms (temporal neocortex), and visceral sensations or automatisms (amygdala, hippocampus).19 Impaired level of consciousness has been proposed to indicate spread to upper brainstem and medial thalamus and subsequent inhibition of bilateral frontoparietal association cortices.20 Secondary generalization has been attributed to spread to corticothalamic networks.3 TLE patients may also have reproductive endocrine abnormalities attributed to connections with the hypothalamus.21
Figure 2–3.
Networks involved in seizure generation in MTLE (initiating circuit), spread, and secondary generalization. MTLE, mesial temporal lobe epilepsy; SNR, substantia nigra pars reticulata. (Modified from Schwarcz R, Scharfman HE, Bertram EH. Temporal lobe epilepsy: renewed emphasis on extrahippocampal areas. In: Davis KL, Charney D, Coyle JT, Nemeroff C, (eds), Neuropsychopharmacology: The Fifth Generation of Progress. Lippincott Williams & Wilkins, 2002; pp. 1843–1855.)

The electrographic signature of typical absences is the generalized or frontally maximal SW complexes. The ictal behavior (absence seizures) consists of sudden behavioral arrest with loss of consciousness and rapid return to baseline toward the end of the ictus, without postictal confusion. Several lines of evidence from electrophysiological or functional neuroimaging studies support the involvement of the corticothalamic pathways in the generation of SW, as originally proposed by Jasper and Kershman,22 although it has been debated whether the origin lies in the cortex or the thalamus.5,7,23 Gloor7 proposed that SW complexes are generated in the context of mild state of cortical hyperexcitability that increases the cortical responsiveness to excitatory thalamic volleys, which would otherwise produce spindle oscillations. In favor of the cortical origin of the SW are the occasional focality of these discharges with rapid bilateral synchrony; the ability of cortex to generate SW and absences in athalamic cats and monkeys; and the finding that thalamic excitation can produce oscillations but not SW.24 In the penicillin model of SW seizures, SW activity was first noted in the cortex.25 However, reports of SW generation following midline thalamic nucleus activation have been reported.5 A SW complex consists of an early spike, corresponding to the cortical activation and a subsequent period of cortical inhibition (slow wave). Bursts of cortical activation (spike) excite the thalamic relay neurons, which depolarize, as well as the GABAergic interneurons in the NRT, which fire in bursts. The NRT neurons then directly inhibit the thalamic relay neurons leading to a fast GABAA-driven IPSP and a slow GABAB-driven IPSP. The hyperpolarization of thalamic neurons activates hyperpolarization-activated cation channels (Ih) and subsequently the low threshold calcium channels (T-channels) leading to a calcium spike that drives action potentials. This in turn activates both cortical neurons and NRT interneurons, leading to the next cycle.5 In classical childhood absence, the SW frequency is around 3 Hz, but more rapid frequencies occur in juvenile absence (>3.5 Hz) and slower (<2.5 Hz) in atypical absences of the Lennox–Gastaut syndrome. It is not unusual that a fast rhythmic activity may precede the SW, reflecting the oscillatory spindle-like activity of the corticothalamic network, driving the SW.
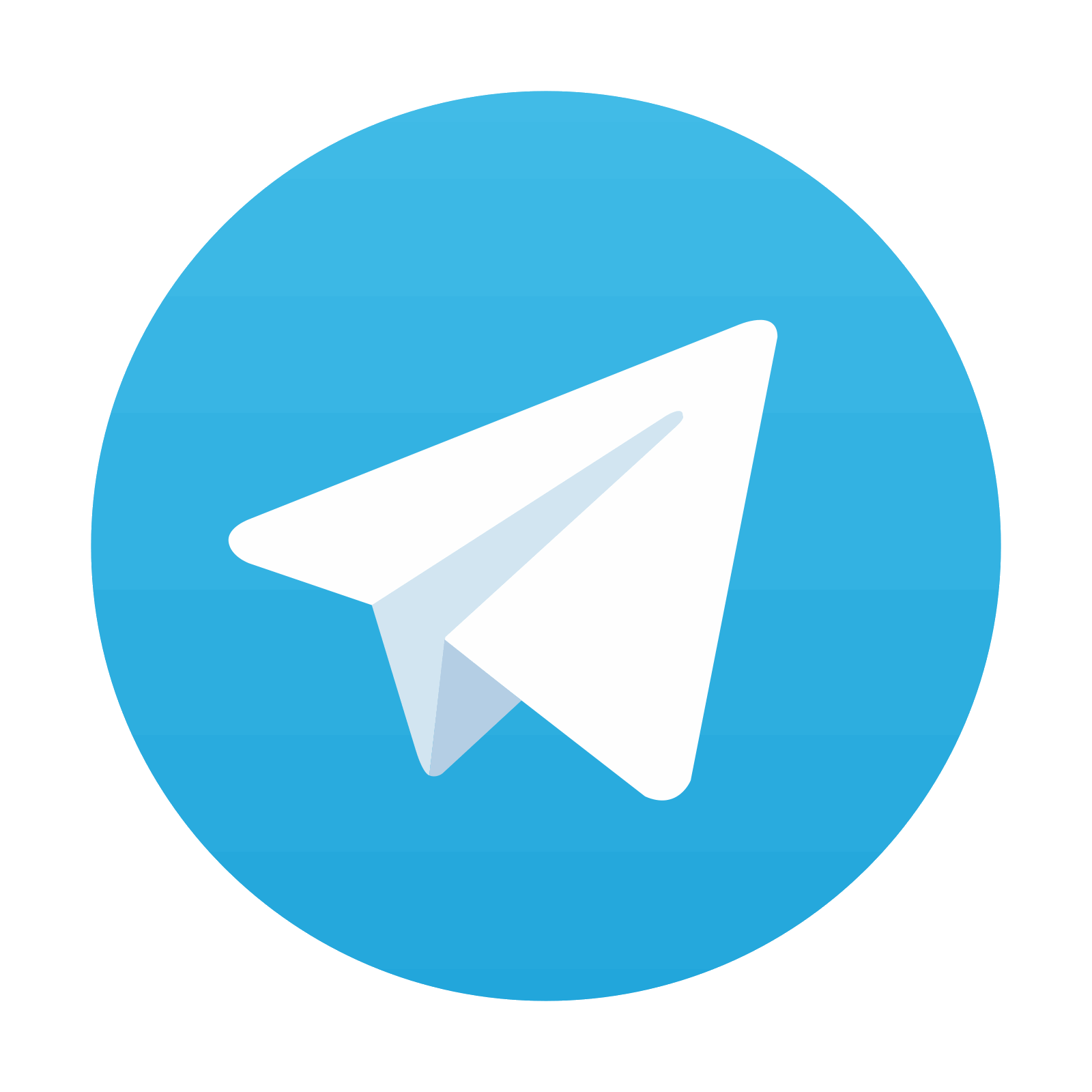
Stay updated, free articles. Join our Telegram channel
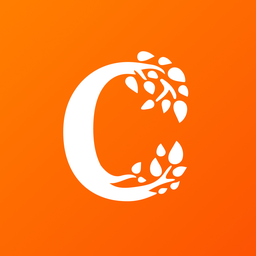
Full access? Get Clinical Tree
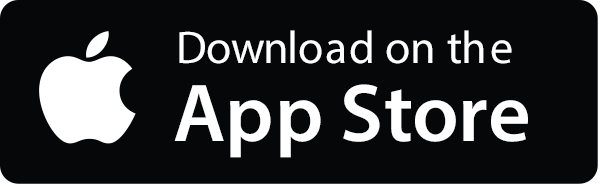
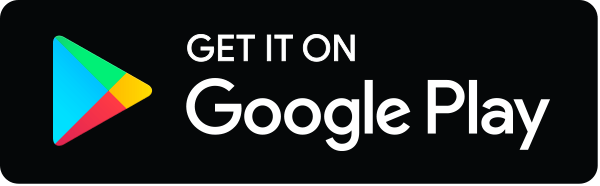