Abstract
The central neuroaxis develops from a streak of ectodermal cells on the dorsal surface of the embryo that specialize into neuroepithelium, starting as a plate of cells that develops into the neural tube. Midline neural tube closure (raphism) starts around the level of the future foramen magnum and proceeds anteriorly forming the future prosencephalon and caudally (primary neurulation) forming the spinal cord above the sacral level. Secondary neurulation follows and results in formation of the conus medullaris, cauda equina, and elements of the hindgut and genitourinary system. Arrested or disturbed development of these neural events are intimately connected to disturbances in formation of overlying mesodermal (muscle, bone, dura) and nonneural ectoderm (cutaneous) structures. The resulting spectrum of malformations includes those due to craniocerebral dysraphism (craniorachischisis, anencephaly, encephaloceles) and those due to spinal dysraphism resulting from disturbances in primary neurulation (myelomeningocele, myeloschisis) and secondary neurulation (meningoceles, lipomeningoceles, tethered cord, other). In recent years, preventive (nutritional) public health strategies and fetal diagnostic advances have significantly reduced the number of live-born infants with spinal dysraphism, while fetal surgical approaches have decreased the rate and severity of complications in live-born infants with these lesions.
Keywords
Primary neurulation, Secondary neurulation, Spina bifida, Anencephaly, Encephalocele, Meningomyelocele, Folate supplementation, Chiari II malformation, Hydrocephalus, Myeloschisis, Meningocele
An understanding of the development of the nervous system is essential for an understanding of fetal and neonatal neurology. An obvious reason for this contention is the wide variety of disturbances of neural development that are flagrantly apparent in the neonatal period and increasingly diagnosed in the fetal period. In addition, all the insults that affect the fetus and newborn, and that are the subject matter of most of this book, exert their characteristic effects in part because the brain is developing in many distinctive ways and at a very rapid rate. As discussed further in Chapter 14 , a strong likelihood exists that many of these common insults exert deleterious and far-reaching effects on certain aspects of neural development—effects that until now have escaped detection by available techniques.
In Chapters 1, 2 , and 4 we emphasize the aspect of normal development that has been deranged, the structural characteristics of the abnormality, and the neurological consequences. It is least profitable to attempt to characterize exhaustively all the presumed causes of these abnormalities of the developmental program. Although a few examples of environmental agents that insult the developing human nervous system at specific time periods and produce a defect are recognized, few of these agents leave an identifying stamp. This obtains particularly because, in the first two trimesters of gestation, the developing brain is not capable of generating the glial and other reactions to injury that serve as useful clues to environmental insults that occur at later time periods. The occasional example of a virus, chemical, drug, or other environmental agent that has been shown to produce a disorder of brain development is mentioned only in passing. However, we emphasize genetic considerations whenever possible because of their importance in parental counseling. Therefore the organizational framework is the chronology of normal development of the human nervous system. A brief review of the major developmental events that occur most prominently during each time period is presented, followed by a discussion of the disorders that result when such development is deranged.
This chapter is devoted to the first major process in human brain development: the formation of the neural tube. These early events culminate in formation of the fundamental central neuroaxis. Development of the neural tube and the subsequent development of the prosencephalon (discussed in the next chapter) can be considered the neural components of embryogenesis. In subsequent chapters we discuss later fetal developmental events that lead to the intrinsic structural development of the central nervous system (CNS).
Normal Development of the Fundamental Central Neuroaxis
Developments in recent years have required a reevaluation of conventional paradigms for developmental disorders of the central neuroaxis. A variety of traditional classification systems have been unsatisfactory, and the persistent inconsistent use of terminology has compromised the diagnostic and prognostic accuracy in clinical practice. New insights from animal models, advanced fetal imaging, and fetal intervention trials for neural tube disorders have led to the notion of primary and secondary consequences of these conditions. For example, the unifying hypothesis for open neural tube defects proposes that the primary failure of spinal closure leads to cerebrospinal fluid (CSF) leakage, which in turn leads to the secondary consequences of posterior fossa underdevelopment, hindbrain herniation, and the development of hydrocephalus. More precise diagnostic paradigms consider the germ cell layers involved (i.e., ectodermal, neuroectodermal, or mesodermal) in the primary dysgenetic lesion, as well as the secondary effects of trauma, toxicity , and mechanical disruption of subsequent development. For example, it is now proposed that the primary defect in anencephaly is likely not failure of neurulation but rather failure of normal cutaneous and mesenchymal development (skin, bone and dural coverings), with a secondary degeneration of the exposed neural tissue. Similarly, lesions such as meningoceles with no neural involvement are often still classified as “closed neural tube defects,” although their etiology is unrelated to neurulation.
Milestones of Major Events
The major developmental events and their peak times of occurrence are shown in Table 1.1 . The time periods are those during which the most rapid progression of the developmental event occurs. Although some overlap exists among these time periods, it is valid and convenient to consider the overall maturational process in terms of a sequence of individual events. In a discussion of the timing of the disorders, the time periods shown in Table 1.1 are obviously of major importance. Nonetheless, it is necessary to recognize that an aberration of a developmental event need not be caused by an insult impinging at the time of the event. Thus a given malformation may not have its onset after the developmental event is completed, but the developmental program may be disturbed at any time before the event is under way. The concept of a termination stage refers to the time in the development of an organ after which a specific malformation cannot occur by any teratogenic mechanism. Thus, in the discussion of timing of malformations, we state that the onset of a given defect could occur no later than a given time. Note that in this text the timing of events is based on the postconceptional (p/c) age, rather than the postmenstrual or gestational age.
MAJOR DEVELOPMENTAL EVENT | PEAK TIME OF OCCURRENCE |
---|---|
Primary neurulation | 3–4 weeks of gestation |
Prosencephalic development | 2–3 months of gestation |
Neuronal proliferation | 3–4 months of gestation |
Neuronal migration | 3–5 months of gestation |
Organization | 5 months of gestation to years postnatally |
Myelination | Birth to years postnatally |
Formation of the Neural Tube
Formation of the neural tube and its coverings proceeds through four phases ( Table 1.2 )—namely gastrulation, primary neurulation, secondary neurulation, and dorsal midline closure of the mesodermal-cutaneous ectodermal layers. Gastrulation is the fundamental formation of the trilaminar plate between days 16 and 18 p/c during which the endodermal, mesodermal, and ectodermal layers of the embryo become distinct. The ectodermal layer differentiates into cutaneous and neural ectoderm. The neural ectoderm develops in the longitudinal plane along the dorsal surface of the embryo as the neural plate. Neurulation refers to the inductive events that occur in the neural plate that result in formation of the neural tube, which ultimately gives rise to the entire CNS. Neurulation can be divided into primary neurulation (i.e., formation of brain and spinal cord down to the sacral level) and secondary neurulation (i.e., events related to the later formation of the sacrococcygeal segments of the spinal cord). With fusion of the neural folds, there is separation of the cutaneous and neural ectoderm, with the ingrowth of the mesodermal layers between them; the timing of these events closely follows the progressive closure of the neural tube. Primary neurulation and secondary neurulation are discussed separately.
MAJOR DEVELOPMENTAL PHASE | PEAK TIME OF OCCURRENCE |
---|---|
1. Gastrulation | 16–18 days p/c |
2. Primary neurulation | 18–26 days p/c |
Neural plate formed | 18 days p/c |
First fusion of neural folds | 22 days p/c |
Anterior neuropore closes | 24 days p/c |
Posterior neuropore closes | 26 days p/c |
3. Secondary neurulation | 26 days p/c—postnatal |
Vacuolation-canalization | 26 days—7 weeks p/c |
Retrogressive differentiation | 7 weeks p/c—postnatal |
4. Disjunction and fusion of mesodermal-cutaneous structures | Tracks regional neural tube closure |
Primary Neurulation
By the end of gastrulation, the most anterior part of the axial mesoderm is the prechordal plate, a critical ventral patterning center for the developing forebrain, while the more posterior parts of the axial mesoderm are made up of the notochord. Primary neurulation is a series of events in the dorsal neural ectoderm of the embryo culminating in formation of the neural tube—rostral to the upper sacral level—and its separation from the other germ cell layers. The critical events are summarized in Box 1.1 . By 18 days p/c the neural plate has been formed by differentiation of the dorsal neural ectoderm from the original ectodermal layer. Next the lateral edges of the neural plate become elevated into neural folds, and the midline of the neural plate invaginates ( Fig. 1.1 ). The neural folds continue to elevate in a dorsomedial direction, until the edges meet in the midline to begin closure of the neural tube. In the human embryo, the first fusion of the neural folds is at the level of the future hindbrain-cervical junction (foramen magnum), which occurs at 22 p/c days. Closure generally proceeds rostrally to form the anterior neural tube (and then the brain) and caudally to form the posterior neural tube (and then the spinal cord), although it is not a simple, zipper-like process. The anterior neuropore of the neural tube closes at approximately 24 days, and the posterior neuropore closes at approximately 26 days, at which point primary neurulation is complete. During closure of the neural tube, cells from the dorsal-most region become separated from the neural tube to form the neural crest, which in turn gives rise to the future craniofacial bony structures, dorsal root ganglia, sensory ganglia of the cranial nerves, autonomic ganglia, Schwann cells, and cells of the pia and arachnoid (as well as melanocytes, cells of the adrenal medulla, and certain skeletal elements of the head and face). Finally, the neural tube becomes physically separated from the meso-endoderm and cutaneous ectoderm, a process called disjunction (see Table 1.2 ). Ongoing interaction between the neural tube and surrounding mesoderm gives rise to the dura and axial skeleton (i.e., the skull and the vertebrae). Understanding this sequence of normal differentiation of the different germ cell layers is critical to an understanding of the different features of cranial and spinal dysraphism.
Peak Time Period
3–4 weeks of gestation
Major Events
Notochord, chordal mesoderm → neural plate → neural tube, neural crest cells
Neural tube → brain and spinal cord → dura, axial skeleton (cranium, vertebrae), dermal covering
Neural crest → dorsal root ganglia, sensory ganglia of cranial nerves, autonomic ganglia, and so forth


Cellular and Molecular Mechanisms of Primary Neurulation.
Primary neurulation occurs under the induction of the underlying notochord and chordal mesoderm during the third and fourth weeks p/c (see Box 1.1 and Fig. 1.1 ). The neural plate deformations required for development of the neural folds, and subsequently the neural tube, are mediated by a variety of cellular and molecular mechanisms, the most important of which involve the cytoskeletal network of microtubules and microfilaments. Under the influence of vertically oriented microtubules, cells of the developing neural plate elongate, while contraction of actin microfilaments arranged circumferentially around the apical portions of the cells results in cells with a broad base and narrow apex. These forces on the neural plate result in invagination of its midline, dorsomedial folding of its edges, and closure to form the neural tube (see Fig. 1.1 ). The process of neural fold bending in a dorsomedial direction also appears to involve differential proliferation and translocation of the neuroepithelial cells. Surface glycoproteins, especially cell adhesion molecules important for cell-cell recognition, as well as adhesive interactions with extracellular matrix, mediate fusion of the opposing neural folds. Other critical molecular events include action of the products of certain regional patterning genes (especially bone morphogenetic proteins and sonic hedgehog), homeobox genes, surface receptors, and transcription factors.
Secondary Neurulation (Caudal Neural Tube Formation)
Secondary neurulation is the process of caudal neural tube formation, which commences at completion of primary neurulation (i.e., on closure of the posterior neuropore around the S2 spinal level, on day 26 p/c; Box 1.2 ). Secondary neurulation occurs in the caudal cell mass and, by forming the remaining sacrococcygeal neural tube, completes neural tube formation. Secondary neurulation gives rise to the conus medullaris, cauda equina, as well as components of the genitourinary tract and hindgut. Starting between 28 and 32 days p/c, the caudal cell mass undergoes vacuolation, coalescence, and canalization, processes that culminate by 7 weeks p/c. At this point the vacuoles connect to the central canal of the neural tube previously formed by primary neurulation. Not infrequently, accessory lumens remain and may be important in the genesis of certain anomalies of neural tube formation (discussed later). Following canalization, the caudal cell mass undergoes retrogressive differentiation between 7 weeks p/c and into postnatal life (see Box 1.2 ). At 8 weeks p/c the spinal cord tissue extends the entire length of the spinal column. Subsequent disproportionate growth of the spinal column results in relative ascent of the conus medullaris (which contains the ventriculus terminalis), leaving the filum terminale in its wake. As a result the conus ascends to the level of L3 by 40 weeks, reaching its final level of L1–L2 by 3 months postnatal.
Peak Time Period
Canalization: 4–7 weeks of gestation
Retrogressive differentiation: 7 weeks of gestation to after birth
Major Events
Canalization: undifferentiated cells (caudal cell mass) → vacuoles → coalescence → contact central canal of rostral neural tube
Retrogressive differentiation: regression of caudal cell mass → ventriculus terminalis, filum terminale
Disorders of Craniospinal Development
The terminology used to describe embryonic anomalies of craniospinal development is inconsistent and often imprecise, which in turn has compromised diagnosis and counseling. To remedy this situation, we first review the definitions and categorization to be used in this text. The term dysraphism is best understood by considering its root (i.e., raphe), which is defined as a line of union between two contiguous bilaterally symmetric structures. Dysraphism is therefore a failure of this process, and in its broadest sense includes any incomplete midline closure of the developing head and spine, and may involve the mesenchymal and ectodermal structures individually or in combination. Embryologically, dysraphic states of the central neuroaxis can be divided into those that occur (1) pre-neurulation (during gastrulation) and involve the neurenteric canal; (2) during primary neurulation, forming the vast majority of open neural tube defects; (3) during secondary neurulation with disturbed development of the caudal cell mass, which is responsible for most closed neural tube defects; or (4) during midline closure of the mesoderm and cutaneous ectoderm. Spina bifida refers only to defects of vertebral arch formation (described later); subtyping of spina bifida is based on the presence and nature of associated neuroectodermal malformations. Isolated vertebral arch dysraphism without underlying neural defects or cystic evagination of the meninges or cord results in true spina bifida occulta .
Neural tube defects refer to a disturbance in neuroectodermal development, defined embryologically as defects of primary or secondary neurulation. Anatomically, neural tube defects can be further categorized by their location relative to the first fusion point of the neural tube, at the level of the future foramen magnum. Lesions of the anterior neural tube (rostral to the foramen magnum) lead to cranial dysraphism, while those of the posterior neural tube (caudal to the foramen magnum) lead to spinal dysraphism. The distinction between open or closed dysraphic lesions is important for understanding the primary lesion and its secondary complications. Open neural tube defects have at least some continuity between the external surface of the fetus and the underlying neural tissue and at least intermittent CSF leakage. In addition, open neural tube defects are usually associated with other CNS anomalies, including hindbrain, callosal, and cerebral cortical malformations. Closed neural tube defects are skin covered, with no exposed neural tissue and no CSF leak; the defect is confined to the spine, and other associated CNS anomalies are rare. As a general rule, most open neural tube defects result from disturbed primary neurulation, while most closed neural tube defects result from disturbed secondary neurulation. However, there are exceptions to this rule. For example, higher (thoracic and cervical) myelomeningoceles may be skin covered (see the discussion of cervical myelocystoceles later on in this chapter), and sacral lesions are occasionally open.
Disorders of primary neurulation are discussed in order of decreasing severity, starting with complete failure of neural tube formation (craniorachischisis totalis), followed by disorders of anterior neural tube formation (cranial dysraphism) and disorders of posterior neural tube formation (spinal dysraphism).
Craniocerebral Dysraphism ( Box 1.3 )
Craniorachischisis Totalis
Anatomical Abnormality.
Craniorachischisis totalis (see Box 1.3 ) results from essentially total failure of neurulation at a very early stage, leaving an exposed neural plate–like structure (with no overlying axial skeleton or dermal covering) running down the entire dorsal extent of the central neuroaxis ( Fig. 1.2 ). Because the neural plate is formed by 18 days p/c, and first point of closure of the neural tube occurs at 22 days p/c, the onset of craniorachischisis totalis is estimated to be no later than 20 to 22 days of gestation. The precise incidence of this lesion is unknown because most cases are aborted spontaneously in early pregnancy, and only a few have survived to early fetal stages.

Anencephaly
Anatomical Abnormality.
Anencephaly (see Box 1.3 and Fig. 1.3 ) has traditionally been classified as an anterior neural tube defect. However, based on human and animal observations, some consider this lesion to be a primary defect in the formation of the cranial vault and its coverings, with secondary degeneration of the cranial neural contents. Specifically, it is proposed that anencephaly results primarily from (partial) absence of the cranial vault (acrania), with initial protrusion of the early fetal brain above the remaining skull bones (exencephaly), and subsequent degeneration of the underlying telencephalic mantle due to direct exposure to the amniotic fluid. According to this viewpoint, anencephaly is not a true neural tube defect, because the primary lesion results from a skeletal (mesodermal) defect. Instead the underlying pathogenetic mechanism of anencephaly invokes a two-hit hypothesis similar to that discussed for myelomeningoceles later in this chapter. This notion that anencephaly is not a true neural tube defect is not universally held. The cranial defect usually involves the frontal bones above the supraciliary ridge, the parietal bones, and the squamous part of the occipital bone, and in the most severe cases, the cranial vault abnormality extends back to or through (holoacrania or holoanencephaly) the foramen magnum. Defects stopping short of the foramen magnum are known as meroacrania or meroanencephaly. The underlying neural tissue defect in anencephaly most commonly involves the forebrain and variable amounts of upper brain stem ( Fig. 1.4 ; see also Fig. 1.3 ), leaving a residual degenerated mass of hemorrhagic, fibrotic, and neuroglial tissue with little definable structure. Onset of anencephaly is estimated to be no later than 24 days of gestation. Polyhydramnios is a frequent feature.


Timing and Clinical Aspects.
Approximately 75% of anencephalic infants are stillborn, and the remainder die in the neonatal period. The disorder is not rare, and epidemiological studies reveal striking variations in prevalence as a function of geographical location, sex, ethnic group, race, season of the year, maternal age, social class, and history of affected siblings. The risk increases with decreasing social class and with a history of affected siblings in the family. Since the late 1970s, the incidence of anencephaly in the United States, like that of myelomeningocele (discussed later), declined to approximately 0.2 per 1000 live births in 1989, remaining relatively stable since then, with the most recent estimates (2009–2011) being 0.28/1000 live births. Both genetic and environmental influences appear to operate in the genesis of anencephaly (see later discussion of myelomeningocele). Recently, maternal use during pregnancy of the selective serotonin reuptake inhibitor paroxetine has been implicated in the development of anencephaly.
Diagnosis.
The skull bones begin to ossify around 11 weeks p/c, making the cranial defect readily identifiable by second trimester fetal ultrasound. In fact, the proportion of anencephaly detected prenatally has been reported to be as high as 96% to 100%. Systematic prenatal detection and elective termination of pregnancy complicated by anencephaly have resulted in a sharp decline in the number of live-born cases.
Encephaloceles
Anatomical Abnormality.
Encephaloceles (see Box 1.3 ) were previously considered a restricted disorder of neurulation involving anterior neural tube closure, although the precise pathogenesis of these lesions remains unknown. It may be better to consider these lesions as developmental disorders of cranial mesoderm in which the cranial defect is associated with a cystic extracranial extension of meninges, neural tissue, and CSF. The notion that encephaloceles are not disorders of primary neurulation is supported by the fact that the herniated brain parenchyma is relatively normal and shows no evidence of defective neurulation. Many encephaloceles are skin-covered (i.e., closed ) lesions. When the cystic lesion includes parts of the ventricular system, the term meningoencephalocystocele is used. Lesions that involve primarily or only the overlying meninges or skull, without obvious inclusion of neural elements, are called cranial meningoceles ( Fig. 1.5 ); these are thought to be later in onset and account for up to 20% of cystic occipital lesions. Encephaloceles occur most commonly (70%–80%) in the occipital region ( Fig. 1.6 ), less commonly in the frontal region ( Fig. 1.7 ), and least commonly in the temporal and parietal regions. Frontal encephaloceles may protrude into the nasal cavity (frontoethmoidal encephaloceles). In the typical occipital encephalocele, the protruding brain is usually derived from the occipital lobe and may be accompanied by dysraphic disturbances involving cerebellum and superior mesencephalon. The neural tissue in an encephalocele usually connects to the underlying CNS through a narrow neck of tissue. The protruding mass is usually represented by a closed neural tube with cerebral cortex, exhibiting a normal gyral pattern, and subcortical white matter. As many as 50% of cases are complicated by hydrocephalus. Encephaloceles located in the low occipital (below the inion) or high cervical regions and combined with deformities of lower brain stem and of base of skull and upper cervical vertebrae characteristic of the Chiari type II malformation (associated with myelomeningocele [discussed later]) comprise the Chiari type III malformation. This type of encephalocele contains cerebellum in virtually all cases and occipital lobes in approximately one-half of cases ( Fig. 1.8 ). Partial or complete agenesis of the corpus callosum occurs in two-thirds of cases. Venous structures may be included in the cyst, and anomalous venous drainage (aberrant sinuses and deep veins) is present in about one-half of patients and may complicate the surgical approach to these lesions.




Timing and Clinical Aspects.
Onset of the most severe lesions is probably no later than the approximate time of anterior neural tube closure (24 days), or shortly thereafter. Infants with encephaloceles not uncommonly exhibit associated malformations. A frequently co-occurring CNS anomaly is subependymal nodular heterotopia. The most commonly recognized syndromes associated with encephalocele are Meckel syndrome (characterized by occipital encephalocele, microcephaly, microphthalmia, cleft lip and palate, polydactyly, polycystic kidneys, ambiguous genitalia, and other deformities) and Walker-Warburg syndrome (see Chapters 6 and 33 ). These disorders, as well as several other less common syndromes associated with encephalocele, are inherited in an autosomal recessive manner. Maternal hyperthermia between 20 and 28 days of gestation has been associated with an increased incidence of occipital encephalocele, as well as with other neural tube defects (discussed later).
Diagnosis.
Skin-covered encephaloceles have normal maternal serum and amniotic fluid AFP levels. Diagnosis by fetal ultrasonography in the second trimester has been well documented. As with myelomeningoceles, open encephaloceles are often associated with decreased extraaxial CSF spaces by fetal imaging. Diagnosis before fetal viability has been followed by elective termination; later diagnosis may require delivery by cesarean section.
Management and Outcome.
Neurosurgical intervention is indicated in most patients. Exceptions include those with massive lesions and marked microcephaly. Surgery is necessary in the neonatal period for ulcerated lesions that are leaking CSF. An operation can be deferred if adequate skin covering is present. Preoperative evaluation has been facilitated by the use of computed tomography (CT) and especially magnetic resonance imaging (MRI) scans. The outcome is difficult to determine precisely because of variability in selection for surgical treatment. In a combined surgical series of 40 infants, 15 infants (38%) died, many of whose complications can be managed more effectively now in neurosurgical facilities. Of the 25 survivors, 14 (56%) were of normal intelligence, although often with motor deficits, and 11 (44%) exhibited both impaired intellect and motor deficits. Not surprisingly, prognosis varies inversely with the extent of herniated neural tissue, with cranial meningoceles (i.e., no obvious neural tissue in the cyst) having the best prognosis. Outcome is more favorable for infants with anterior encephaloceles than those with posterior encephaloceles. Thus, in one series of 34 cases, mortality was 45% for infants with posterior defects and 0% for those with anterior defects. Normal outcome occurred in 14% of the total group with posterior defects and in 42% of those with anterior defects.
Spinal Dysraphism
In the following we first consider disorders of primary neurulation, usually open neural tube defects, because these originate earlier in embryonic development and are by far the most common and clinically relevant spinal dysraphic conditions. Thereafter we discuss disorders of secondary neurulation, which more commonly result in closed neural tube defects.
Disorders of Primary Neurulation in the Spine
There are two major disorders of primary neurulation above the sacral level, namely, myelomeningocele and myeloschisis. The essential difference between these two lesions is that a cystic component is present in myelomeningocele (at least initially), consisting of dysplastic meningeal tissue usually leaking CSF, whereas in myeloschisis the neural placode is completely exposed, with no cystic meningeal or cutaneous covering and often continuous CSF leakage from the spinal central canal. Myelomeningoceles are usually displaced in a dorsal direction beyond the level of the surrounding skin, by a collection of CSF ventral to the cord ( Fig. 1.9 ). Conversely, myeloschisis lesions are usually adherent to the anterior wall of the vertebral canal and are therefore either flush with, or below, the plane of the surrounding skin (discussed later). The terminology used for both these lesions has been inconsistent. Some authors use the term myelomeningocele for cystic lesions that contain abnormal neural tissue, even if the cystic lesion is skin covered (i.e., a closed defect). The term myeloschisis has also been applied inconsistently, with some authors using the term for open lesions extending down most of the neural tube, a lesion that others refer to as rachischisis. Myelomeningoceles are the most common open dysraphic lesion of the spinal cord by far, have been subject to the most investigation, and have shown some response to medical and surgical intervention (discussed later). In addition, most cases of myelomeningocele now survive. For these reasons, the following discussion focuses largely on myelomeningocele.

Myelomeningocele
Anatomical Abnormality.
The essential neural defect in myelomeningocele is restricted failure of primary neurulation with varying degrees of posterior neural tube closure. Approximately 80% of lesions occur in the lumbar (thoracolumbar, lumbar, lumbosacral) area, presumably because this is the last area of the neural tube to close. Myelomeningoceles are represented by a neural plate or abortive neural tube–like structure (placode) in which the ventral half of the cord is relatively less affected than the dorsal. Most of the lesions are associated with dorsal protrusion of the neural tissue, such that a sac is created on the back ( Figs. 1.10 and 1.11 ; see also Fig. 1.9 ). Bony effects of the overlying tissue include failure (lack of fusion or an absence) of vertebral arch development (spina bifida), with bilateral broadening of the vertebrae and lateral displacement of pedicles, leading to a widened vertebral canal. The caudal extent of the vertebral changes is usually considerably greater than the extent of the neural lesion. Soft-tissue covering consists of a porous cystic sac of dysplastic meningeal tissue and a deficient skin cover.


Cervical myelomeningoceles are rare and differ in embryology, prognosis, and management from myelomeningoceles at lower spinal levels (discussed later). These lesions are cystic and meet criteria as myelomeningoceles because they contain neuroglial tissue and CSF. However, unlike lower myelomeningoceles, the spinal cord is normal or near normal and remains within the spinal column. A stalk of neuroglial of fibrovascular tissue extends through a small midline gap between the dorsal columns of the spinal cord, passes through the bony spinous defect, and across the CSF-filled cyst to attach to the cyst wall, thereby tethering the spinal cord. Unlike most other myelomeningoceles, these cervical lesions are almost always covered by full-thickness skin (i.e., closed ) without CSF leakage. Cervical myelomeningoceles are commonly associated with occult spinal lesions in other areas, such as hemivertebra, syringomyelia, and diastematomyelia (discussed later). Chiari II lesions are common in cervical myelomeningoceles, but intracranial neural lesions commonly associated with more caudal myelomeningoceles (such as fused thalami, callosal anomalies, and cortical malformations) are rare.
Timing.
The onset of a typical myelomeningocele is probably no later than 26 days of gestation, when the posterior neuropore closes.
Etiological Considerations.
Prevention of myelomeningocele and other neural tube defects necessitates understanding of their causes. Recognized causes of such defects include (1) multifactorial inheritance, (2) single mutant genes (e.g., the autosomal recessively inherited Meckel syndrome), (3) chromosomal abnormalities (e.g., trisomies of chromosomes 2, 7, 9, 13, 14, 15, 16, 18, and 21 and duplications of chromosomes 1, 2, 3, 6, 7, 8, 9, 11, 13, 16, 20, and X), (4) certain rare syndromes of uncertain modes of transmission, (5) specific teratogens (e.g., aminopterin, thalidomide, valproic acid, carbamazepine), and (6) specific phenotypes of unknown causes (e.g., cloacal exstrophy and myelocystocele). In rodent models of neural tube defects, more than 240 gene mutations have been identified. Recent human studies suggest that a variety of genetic mutations may play a role, likely through complex polygenic interactions, possibly with superimposed epigenetic influences to produce the different neural tube defect phenotypes. Of defects resulting from these causes, most cases (≈80%) are encompassed within the group in which the neural tube defect is the only major congenital abnormality and inheritance is multifactorial (i.e., dependent on a genetic predisposition that is polygenic and influenced by minor additive genetic variations at several gene loci). Overall, genetic factors are thought to play a role in 60% to 70% of these cases. Environmental influences may play an important role on this substrate. Less than 10% of neural tube defects are part of syndromic conditions.
Factors establishing the combined influence of both genetic and environmental influences are summarized in Tables 1.3 and 1.4 . Factors establishing the genetic role include (1) a preponderance in female patients, (2) ethnic differences that persist after geographical migration, (3) increased incidence with parental consanguinity, (4) increased rate of concordance in apparently monozygotic twin pairs, and (5) increased incidence in siblings (as well as in second-degree and, to a lesser extent, third-degree relatives) and in children of affected patients. The possibility of important environmental influences is suggested particularly by large variations in incidence as a function of geographical location and time period of study (see Table 1.3 ). Particularly potent data to suggest environmental influences relate to long-term trends in incidence. For example, in the northeastern United States, an epidemic period could be defined between approximately 1920 and 1949, with a peak between 1929 and 1932. Since the late 1980s, a prominent steady decline in incidence has occurred in both the United States and Great Britain (see earlier). a
a References .
The interaction of environmental and genetic influences has been demonstrated in experimental studies of the curly-tail mouse, in which a neural tube defect is inherited as an autosomal recessive trait. Among specific environmental influences , a particularly important role for folate deficiency during the period of neural tube formation is suggested by experimental and clinical studies (discussed later). Other environmental factors, such as prenatal exposure to maternal hyperthermia, maternal diabetes mellitus, valproic acid (see Chapter 38 ), carbamazepine (see Chapter 38 ), maternal obesity, and low maternal vitamin B 12 concentrations, also are of varying importance (see Table 1.4 ).FACTOR | TIME PERIOD | PREVALENCE |
---|---|---|
Country | ||
England/Wales | 1996 | 0.32 |
Finland | 1996 | 0.41 |
Norway | 1996 | 0.57 |
Northern Netherlands | 1996 | 0.63 |
Region of country | ||
Northern China | 1992–1993 | 2.92 |
Southern China | 1992–1993 | 0.26 |
Time period | ||
Eastern Ireland | 1980 | 2.7 |
Eastern Ireland | 1984 | 0.6 |
Ethnic/racial (California) | ||
Non-Hispanic white | 1990–1994 | 0.47 |
Hispanic | 1990–1994 | 0.42 |
African American | 1990–1994 | 0.33 |
Asian | 1990–1994 | 0.20 |
Prenatal diagnosis and elective termination (England/Wales) | ||
Live and stillbirths | 1996 | 0.09 |
Live and stillbirths and terminations | 1996 | 0.31 |
FACTOR | RELATIVE RISK |
---|---|
Previous affected pregnancy (same partner) | 30 |
Inadequate intake of folic acid | 2–8 |
Pregestational diabetes | 2–10 |
Intake of valproic acid or carbamazepine | 10–20 |
Low vitamin B 12 | 3 |
Obesity | 1.5–3.5 |
Hyperthermia | 2 |
The increased incidence of neural tube defects in siblings of index cases has had major importance for genetic counseling. Recurrence risks for neural tube defects are around 3% after a single affected pregnancy, increasing to 12% after two affected pregnancies. However, precise estimates of risks in subsequent siblings must take into account the population under study (see Table 1.3 ). A striking relationship between recurrence risk and the level of the myelomeningocele in the index case has been shown. Thus the risk for recurrence in a sibling was 7.8% if the index case had a lesion at T11 or above, but only 0.7% if the lesion was below T11. A decline in the risk of neural tube defect as birth order increases also has been defined ; for example, in a study in Albany, New York, the risk for subsequent affected siblings (1.4%) was significantly less than for previously affected siblings (3.1%).
Maternal diabetes is associated with a threefold increase in birth defects, but this risk increases to 5.4-fold for neural tube defects and 170-fold for caudal cell mass dysplasias (discussed later). At highest risk are pregnancies complicated by hyperglycemia around the time of conception (pregestational diabetes). Pregestational obesity is also a risk factor for neural tube defects. In one large population-based study, there was a fourfold increase in neural tube defects in women with body mass index (BMI) greater than 40. Other studies have shown a significantly increased risk for neural tube defects when maternal pregestational BMI exceeds 30. The association between neural tube defects and obesity is significantly stronger for spina bifida than for anencephaly. Recent reports suggest that obesity-related neural tube defects are folate unresponsive.
Clinical Aspects.
The precise incidence of myelomeningocele is difficult to establish, in part because of differences in the terminology used in reports. The populations included in these studies are variable, with some including anencephaly, some only open neural tube defects, or some with a broad spectrum of neural tube defects. Although there is a wide variation between and within countries, it is generally agreed that the incidence of neural tube defects has been decreasing in developed countries for the past three decades, even before the advent of folic acid supplementation (discussed later). a
a References .
Factors implicated in this decline include dietary folate supplementation, improved antenatal diagnosis and, in some areas, elective termination. Nonetheless, the global burden of neural tube defects (mostly anencephaly and myelomeningocele) remains high and estimated to exceed 300,000 cases per year, with highest prevalences in low- and middle-income populations. At the same time, there has been a steady increase in the survival of myelomeningocele, with one study reporting survival rates of 71%, 69%, and 66% at 1, 10, and 20 years, respectively.Neurological Features.
The clinical features of myelomeningocele relate primarily to the nature of the primary spinal lesion, as well as the presence and severity of associated complications, such as hindbrain herniation, hydrocephalus, and other intracranial developmental lesions.
The disturbances of neurological function, of course, depend on the level of the spinal lesion. Particular attention should be paid to examination of motor, sensory, and sphincter function. Moreover, in the first days of life, motor function subserved by segments caudal to the level of the lesion is common but then generally disappears after the first postnatal week. Table 1.5 lists some of the important correlations among motor, sensory, and sphincter function, reflexes, and segmental innervation. Assessment of the functional level of the lesion allows reasonable estimates of potential future capacities. Thus most patients with lesions below S1 ultimately are able to walk unaided, whereas those with lesions above L2 usually are wheelchair dependent for at least a major portion of their activities. Approximately one-half of patients with intermediate lesions are ambulatory (L4, L5) or primarily ambulatory (L3) with braces or other specialized devices and crutches. Considerable variability exists between subsequent ambulatory status and apparent neurological segmental level, especially in patients with midlumbar lesions. Good strength of iliopsoas (hip flexion) and of quadriceps (knee extension) muscles is an especially important predictor of ambulatory potential rather than wheelchair dependence. Deterioration to a lower level of ambulatory function than that expected from segmental level occurs over years, and this tendency is worse in the absence of careful management. In addition, patients with lesions as high as thoracolumbar levels, at least as young children, can use standing braces or other specialized devices to be upright and can be taught to swivel walk . Indeed, continuing improvements in ambulatory aids and their use are constantly increasing the chances for ambulation in children with higher lesions (see Results of Therapy). Segmental level also is an important determinant of the likelihood of the development of scoliosis. Most patients with lesions above L2 ultimately exhibit significant scoliosis, whereas this complication is unusual in patients with lesions below S1.
MAJOR SEGMENTAL INNERVATION a | MOTOR FUNCTION | CUTANEOUS SENSATION | SPHINCTER FUNCTION | REFLEX |
---|---|---|---|---|
L1–L2 | Hip flexion | Groin (L1) | — | — |
Anterior, upper thigh (L2) | ||||
L3–L4 | Hip adduction | Anterior, lower thigh and knee (L3) | — | Knee jerk |
Knee extension | Medial leg (L4) | |||
L5–S1 | Knee flexion | Lateral leg and medial foot (L5) | — | Ankle jerk |
Ankle dorsiflexion | Sole of foot (S1) | |||
Ankle plantar flexion | ||||
S1–S4 | Toe flexion | Posterior leg and thigh (S2) | Bladder and rectal function | Anal wink |
Middle of buttock (S3) | ||||
Medial buttock (S4) |
a Segmental innervation for motor and sensory functions overlaps considerably; correlations shown are approximate.
Cervical myelomeningoceles are almost always associated with a normal neurological outcome if surgical detethering is performed in infancy. Significant primary motor, sensory, bowel, and bladder deficits are rare. Delayed or incomplete surgical detethering may result in progressive neurological deficits over time.
Associated Anomalies and Putative Mechanisms
Chiari Type II Malformation.
The Chiari II malformation ( Fig. 1.12 ) is a virtually ubiquitous complication of thoracolumbar, lumbar, and lumbosacral myelomeningocele. The central feature of this complex anomaly is a small posterior fossa, the contents of which are crowded and distorted. A Chiari II malformation involves the midbrain and hindbrain (pons, medulla, and cerebellum) and cervical spinal cord and its components and associations are listed in Box 1.4 . Features of the Chiari II malformation may be explained by the unified theory , in which the ongoing spinal CSF leak results in failure of CSF support of the ventricles, including the fourth ventricle, which in turn leads to an underdeveloped small posterior fossa (with a low-set torcular and incomplete bony growth). The principles of the unified theory are supported by studies of fetal myelomeningocele repair showing reduced hindbrain herniation and reduced brain stem compression. The degree of hindbrain herniation does not appear to be related to the level of the spinal lesion. However, some degree of hindbrain herniation is almost universally present in open myelomeningocele and remains the leading cause of death in the first 5 years of life. The cerebellar hemispheres are underdeveloped, but the cerebellar dysmorphology does not affect all cerebellar regions equally. Compared with controls and corrected for reduced global volume, the anterior lobe is enlarged while the posterior lobe is reduced in size. Absence of brain stem nuclei, including the basal pontine and olivary nuclei, are also seen; these nuclei and the cerebellum have a common origin in the alar plate of the rhombencephalon.

Chiari II Malformation
Ubiquitous with lumbar-sacral myelomeningoceles
Temporal Features
Usually present by 2nd trimester
Etiological Features
Small posterior fossa is the fundamental mechanism; due to CSF leak from spinal lesion
Crowded and distorted contents
Anatomic Features
Small posterior fossa
Low-set torcular caudal herniation through foramen magnum (medulla, 4th ventricle, inferior vermis)
Rostral herniation through the tentorial notch (superior vermis)
Pressure/traction effects
Elongation and thinning of upper medulla and pons
Persistence of embryonic flexure
Cerebellar hemispheres may wrap around brain stem
Hydrocephalus due to disturbed flow dynamics from aqueduct, 4th ventricle, and subarachnoid space compression
Bony defects of foramen magnum, occiput and upper cervical vertebrae
Associated features of uncertain pathogenesis
Cerebellar dysplasia, hypoplasia or agenesis
Significant reduction of Purkinje cells
Absence of brain stem nuclei (basal pontine, olivary, other)
Clinical Importance
Stridor, apnea, cyanotic spells, and dysphagia may develop
Role in development of hydrocephalus
Hydrocephalus
Develops in 85%–90% of lumbar-sacral myelomeningoceles
Temporal Features
Most rapid progression occurring in first postnatal month
Dilation of ventricles before rapid head growth or before signs of increased intracranial pressure or both
Etiological Features
Chiari type II with obstruction of fourth ventricular outflow
Aqueductal stenosis
Impaired CSF flow through narrowed subarachnoid spaces and crowded posterior fossa
Importance
Requirement for shunt and its complications (especially infection) are a major cause of neurologic morbidity.
The Chiari type II malformation is responsible for both brain stem dysfunction (a serious complication in a minority of patients with myelomeningocele) and hydrocephalus, a serious complication in most patients with myelomeningocele (see earlier). Hydrocephalus associated with the Chiari type II malformation probably results primarily from one or both of two basic causes (see Box 1.4 ). The first is the hindbrain malformation that blocks either the fourth ventricular CSF outflow or the CSF flow through the posterior fossa. The second is at the aqueductal level, with aqueductal stenosis present in approximately 40% to 75%, and aqueductal atresia in an additional 10% of cases with Chiari II malformations. Studies of human embryos and fetuses with myelomeningocele support the concept that the Chiari type II hindbrain malformation is a primary defect and not a result of hydrocephalus.
Moreover, studies of a mutant mouse with defective neurulation ( Splotch ) provide insight into the mechanism by which myelomeningocele may lead to the Chiari type II malformation. Thus, in this model, it is clear that the Chiari type II malformation results from growth of the hindbrain in a posterior fossa that is too small. Hydrocephalus then results from the Chiari type II malformation, as described earlier. Additional support for this formulation is the demonstration that closure of the myelomeningocele in the second trimester of fetal life, before the most rapid growth of the cerebellum, results in upward displacement of the inferiorly herniated cerebellar vermis, expansion of the posterior fossa, improvement in CSF flow, and reduced need for ventriculoperitoneal shunting for hydrocephalus (discussed later; see Fig. 1.10 ).
Clinical features directly referable to the hindbrain anomaly of the Chiari type II malformation (i.e., not to hydrocephalus) are probably more common than is recognized. In one carefully studied series of 200 infants, one-third exhibited feeding disturbances (associated with reflux and aspiration), laryngeal stridor, or apneic episodes (or all three). In one-third of these affected infants, death was “directly or indirectly attributed to these problems.” Indeed, in this and similar series, at least one-half of the deaths of infants with myelomeningocele can be attributed to the hindbrain anomaly (despite treatment of the back lesion and hydrocephalus). In a cumulative series of 142 infants, the median age at onset of symptoms referable to brain stem compromise was 3.2 months. The clinical syndromes of brain stem dysfunction and their relation to mortality are presented in Table 1.6 . The 19 affected infants represented 13% of those with myelomeningocele. The principal clinical abnormalities in this and related studies reflect lower brain stem dysfunction and include vocal cord paralysis with stridor, abnormalities of ventilation of both obstructive and central types (especially during sleep), cyanotic spells, and dysphagia. The full constellation of stridor, apnea, cyanotic spells, and dysphagia is associated with a high mortality (see Table 1.6 ). Such sensitive assessments of brain stem function as brain stem auditory-evoked responses, polysomnography, pneumographic ventilatory studies, and somatosensory-evoked responses are abnormal in approximately 60% in infants with myelomeningocele and are the neurophysiological analogues of the clinical deficits.
CLINICAL FEATURES | NUMBER | MORTALITY |
---|---|---|
Stridor | 10 | 0 |
Stridor and apnea | 4 | 25% |
Stridor, apnea, cyanotic spells, and dysphagia | 5 | 60% |
Total | 19 | 21% |
The clinical abnormalities of brain stem function have three primary causes. First, they relate in part to the brain stem malformations, which involve cranial nerve and other nuclei, and are present in most cases at autopsy ( Table 1.7 ). Second, compression and traction of the anomalous caudal brain stem by hydrocephalus and increased intracranial pressure may play a role, especially in the vagal nerve disturbance that results in the vocal cord paralysis and stridor. Third, ischemic and hemorrhagic necrosis of brain stem is often present and may result from the disturbed arterial architecture of the caudally displaced vertebrobasilar circulation.
Total With Brain Stem Malformation | 76% |
Defective myelination | 44% |
Hypoplasia of cranial nerve nuclei | 20% |
Hypoplasia or aplasia of olives | 20% |
Hypoplasia or aplasia of basal pontine nuclei | 16% |
Hypoplasia of tegmentum | 4% |
Hydrocephalus.
Several clinical features are helpful in evaluating the possibility of hydrocephalus. First, on examination, the status of the anterior fontanelle and the cranial sutures should be noted. A full anterior fontanelle and split cranial sutures are helpful signs for the diagnosis of increased intracranial pressure. CSF leakage from the myelomeningocele provides decompression in this situation, and the signs of increased intracranial pressure may be absent or delayed. Evaluation of the head size provides useful information. If the head circumference is more than the 90th percentile, approximately a 95% chance exists that appreciable ventricular enlargement is present. If the head circumference is less than the 90th percentile, an approximately 65% chance of hydrocephalus still exists. The site of the lesion is also helpful in predicting the presence or imminent development of hydrocephalus. With occipital, cervical, thoracic, or sacral lesions, the incidence of hydrocephalus is approximately 60%; with thoracolumbar, lumbar, or lumbosacral lesions, the incidence of hydrocephalus is approximately 85% to 90%.
Signs of increased intracranial pressure are not prerequisites for the diagnosis of hydrocephalus in the newborn and indeed are observed in only approximately 15% of newborns with myelomeningocele. Serial ultrasound scans are important because progressive ventricular dilation, without rapid head growth or signs of increased intracranial pressure, occurs in infants with myelomeningocele in a manner analogous to the development of hydrocephalus after intraventricular hemorrhage (see Chapter 24 ). The most common time for hydrocephalus with myelomeningocele to be accompanied by overt clinical signs is 2 to 3 weeks after birth; more than 80% of infants who have hydrocephalus with myelomeningocele and who do not undergo shunting procedures exhibit such clinical signs by 6 weeks of age.
Other Central Nervous System Anomalies.
Other anomalies of the CNS associated with myelomeningocele and the Chiari II lesion include cerebral cortical anomalies, callosal defects, small posterior fossa, with decreased size of the cerebellum. Perhaps most important of these are abnormalities of cerebral cortical development. In earlier studies, the pathological finding of microgyria was described in 55% to 95% of cases. Whether this finding reflected a true cortical dysgenesis was not clear, but its presence was of major potential importance because of a relationship with the intellectual deficits that occur in a minority of these patients. Moreover, the occurrence of seizures in approximately 20% to 25% of children with myelomeningocele may be accounted for in part by such cortical dysgenesis. This issue was clarified considerably by a careful neuropathological study of 25 cases of myelomeningocele ( Table 1.8 ). Fully 92% of the brains showed evidence of cerebral cortical dysplasia, and 40% had overt polymicrogyria. Thus impaired neuronal migration was a common feature. In addition, callosal anomalies, including hypoagenesis (reduced ventrodorsal extent) and hypoplasia (reduced thickness), are thought to occur to some degree in almost all cases of myelomeningocele. In callosal hypogenesis, the rostrum, splenium, and posterior body are the most commonly missing elements. Even when present, the posterior elements (i.e., isthmus and splenium) are the most commonly hypoplastic regions. Thoracic myelomeningoceles are twofold more likely to have splenial agenesis than lower myelomeningoceles. Up to 44% of myelomeningocele cases have callosal hypoplasia, in which the callosum is present in its entire rostrocaudal extent but is thinned. More recent MRI studies using surface area and diffusion tensor analyses support the notion that the thinner corpus callosum results from fewer crossing axons. Several studies have suggested that volume of the entire corpus callosum, as well as specifically the genu and splenium, correlate with cognitive outcome.
Total with Cerebral Cortex Dysplasia | 92% |
Neuronal heterotopias | 44% |
Polymicrogyria (with disordered lamination) | 40% |
Disordered lamination only | 24% |
Microgyria, normal lamination | 12% |
Profound migrational disturbances | 24% |
Other anomalous features, such as cranial lacunae, hypoplasia of the falx and tentorium, low placement of the tentorium, anomalies of the septum pellucidum, anterior and inferior pointing of the frontal horns, thickened interthalamic connections, and widened foramen magnum, are of uncertain clinical significance. However, they are visualized readily to varying degrees with CT, MRI, and cranial ultrasonography. Midbrain anomalies include tectal beaking , which is due to fusion of the colliculi and is evident in about 65% of cases. Anomalies in position of the cerebellum are observable in utero by ultrasonography or MRI. Cerebellar dysplasia, including heterotopias, is definable neuropathologically in 72% of cases.
Diagnosis.
Maternal serum alpha-fetoprotein (AFP) levels and ultrasonography are the primary screening techniques for the prenatal diagnosis of neural tube defects. AFP is the major protein component of human fetal serum ( Fig. 1.13 ) and can be detected 30 days after conception, peaking at between 10 and 13 weeks of gestation. Current recommendations are for maternal serum AFP testing between 15 and 20 weeks’ gestation. Increased levels of AFP in the amniotic fluid occur with open neural tube defects and anencephaly; the mechanism for the elevated levels is thought to represent transudation of the protein from the membranes covering the lesion (see Figs. 1.9 and 1.11 ). Positive maternal serum AFP testing should be repeated, since false positive tests are not uncommon. Conversely, there are conditions other than open neural tube defects that may elevate maternal serum and amniotic fluid AFP levels, including abdominal wall defects such as omphalocele or gastroschisis. Until the mid-1980s, amniocentesis for detection of elevated levels of AFP in amniotic fluid was the most common, albeit invasive, procedure for suspicion of an open neural tube defect. Several reports in the late 1970s indicated that determination of maternal serum AFP levels was useful for screening for neural tube defects. The largest study involved measurements in more than 18,000 pregnant women in the United Kingdom. The optimal time for measurement was shown to be 16 to 18 weeks of pregnancy. Subsequent experience in Scotland, Sweden, Wales, and the United States confirmed the high sensitivity of the analysis of AFP in serum, and large-scale screening programs are now well established. In situations where the origin of elevated maternal serum AFP remains in question, amniotic fluid levels of AFP should be tested and, if elevated, should be followed by acetylcholinesterase (AChE; primarily neuronal in origin) levels. Elevation of both AFP and AChE in the amniotic fluid confirms a neural origin for the elevated AFP.

The diagnosis and anatomical details of a fetal neural tube defect are confirmed by ultrasonography and MRI. The earliest ultrasonographic features include changes in the configuration of the posterior fossa, in addition to the lesion itself. After 12 weeks’ gestation the cranial markers of myelomeningocele (i.e., banana and lemon signs [ Fig. 1.14 ], decreased extraaxial CSF, and small cisterna magna) become detectable by ultrasound and are useful supportive findings. Fetal MRI is the diagnostic study of choice in the fetal period and best delineates the anatomical details (see Fig. 1.10 ).

Management.
The management of myelomeningocele has undergone major changes in recent decades, both in terms of primary prevention, as well as lesion repair in the newborn, and more recently in the fetus. The following briefly reviews current concepts in management.
Primary Prevention.
Management of the patient with myelomeningocele, or of any patient with a neural tube defect, should begin with the following question: How could this have been prevented? Indeed, prevention must be considered the primary goal for the future. Major advances have been made in this direction. Prenatal diagnosis of neural tube defects and termination of pregnancy involving an affected fetus are effective methods of secondary prevention. However, a method of primary prevention would be more widely acceptable. Evidence now shows that folate supplementation around the time of conception, and therefore neural tube closure, has a major preventive effect on the occurrence of neural tube defects. a
a References .
The effect of multivitamin supplementation ( Box 1.5 ) before and during early pregnancy on recurrence of neural tube defects was first studied definitively by Smithells and colleagues in a recruited series of women with histories of births of one or more previously affected children. The multivitamin supplement contained physiological quantities of vitamins, such as folate, riboflavin, ascorbic acid, and vitamin A. The results of the study were striking. Of 454 women taking supplements, only 3 (0.7%) had recurrences, whereas of 519 women not taking supplements, 24 (4.7%) had recurrences. Although the study was criticized for several methodological issues, the data were promising. A subsequent study by Smithells and coworkers showed a similarly striking effect. A noncontrolled study in the United States on women identified largely by elevated serum AFP levels, measured in a single regional laboratory, confirmed the beneficial role of folate-containing multivitamins. Moreover, the beneficial effect of folate was related clearly to the time during pregnancy when neural tube closure occurs.
- ◆
1959: Failed abortions using an FA antagonist were associated with an increase in NTDs.
- ◆
1965: Embryopathy of maternal FA deficiency includes NTD.
- ◆
1980–1983: MVI supplementation reduced NTD risk.
- ◆
1989: MVI supplementation beneficial effect corroborated.
- ◆
1989: Noncontrolled study of MVI/FA supplementation in early pregnancy leads to decrease in NTD.
- ◆
1991: Medical Research Council (United Kingdom) Vitamin Study. Large RCT of FA supplementation (4 mg/day) in women with previous NTD fetus showed an 83% reduction.
- ◆
1992: RCT of FA supplementation (0.8 mg/day) from 1 month preconception through first 8 weeks of pregnancy reduced the risk of a first NTD-affected fetus.
- ◆
1992: Centers for Disease Control and Prevention (United States) recommended FA supplementation (0.4 mg/day) for all women of childbearing age capable of becoming pregnant in the United States.
- ◆
1997: Despite increased public awareness, supplementation campaign proved disappointing; <1/3 women compliant and >50% of pregnancies in United States are unplanned.
- ◆
1998: Fortification campaign implemented in the United States with goal of ensuring that women of childbearing age received the daily recommended dose of 400 µg/day
- ◆
2002–2003: Several Canadian reports describe a 32%–54% decrease in NTD since dietary FA fortification.
- ◆
2010: United States report ~20% decrease in NTD since dietary FA fortification.
- ◆
2015: Late high-dose (5 mg/day) rescue FA after early pregnancy diagnosis may be protective.
- ◆
Currently: More than 75 countries have mandatory FA fortification programs.
FA , Folic acid; MVI , multivitamins; NTD , neural tube defect; RCT , randomized clinical trial.
After the aforementioned study, the British Medical Research Council completed an extremely important multicenter study ( Box 1.5 ). Women were assigned randomly to four groups allocated to receive one of the following regimens of supplementation: folate and a multivitamin supplementation of “other vitamins,” folate alone, “other vitamins” alone, or no folate or “other vitamins.” The results were decisive in demonstrating the preventive effect and the specific role of folate (vs. other components of the previously used multivitamin preparations). The overall reduction in neural tube defects was 83%. The findings clearly had major implications for the primary prevention of neural tube defects. On the basis of this study, the US Centers for Disease Control and Prevention (CDC) recommended an increase in folic acid intake by 0.4 mg/day for women from the time they plan to become pregnant through the first 3 months of pregnancy. The folate was not recommended to be administered as a multivitamin preparation because of the potential danger for toxicity from excessive amounts of other vitamins in the multivitamin preparation. Because of the uncertainty of the degree of risk from the folate supplementation, the initial recommendations were directed only to women who had had a previous pregnancy complicated by a neural tube defect, as in the British study. Subsequently, two studies showed a preventive effect of periconceptional folic acid exposure on the occurrence of neural tube defects in populations of women without a prior affected child. These observations were followed by the recommendation of the US Public Health Service and the American Academy of Pediatrics that “all women capable of becoming pregnant consume 0.4 mg of folic acid daily to prevent neural tube defects.”
The optimal methods of folate supplementation and dose of the supplement are not totally clarified. Public educational campaigns, albeit useful, have not been entirely successful, especially because as many as 50% of pregnancies are unplanned, and only a few of the nonplanners are reached by such campaigns. In 1998 the US Food and Drug Administration mandated fortification of all enriched grain products with folate (0.14 mg/100 g), which resulted in a 30% decrease in neural tube defects. Similar programs have been instituted in many other countries and have resulted in an approximately 50% reduction in prevalence of neural tube defects. a
a References .
However, the British Medical Research Council study used a 4 mg (rather than 0.4 mg) daily folate dose and achieved an 83% reduction in prevalence of lesions. Thus one expert in the field recommended a public health policy that includes “both the mandatory fortification of flour and a recommendation that all women planning a pregnancy take 5 mg of folic acid per day.”Together these studies have led to the conclusion that folate supplementation was capable of reducing but not eradicating neural tube defects, giving rise to the notion of “folic acid–preventable spina bifida and anencephaly” (FAPSBA). It is estimated that 50% to 72% of neural tube defects are preventable through folate supplementation. The reduction in anencephaly and facial defects has been less striking than that of spina bifida. In addition, in the postfortification era the initial decrease in neural tube defects has been sustained without further decline. In the United States, Hispanics continue to have the highest rate, whereas non-Hispanic blacks have the lowest rate. The decreases in prevalence of neural tube defects following fortification have been associated with increasing levels of serum and red blood cell (RBC) folate levels in the population and an almost complete eradication of folate deficiency. Recent studies have suggested that the majority of FAPSBA in the United States is prevented by fortification; however, more than 20% of women of childbearing age still do not attain the RBC folate levels known to decrease the risk of neural tube defects. This has in turn led to recommendations for strategies that target specific RBC folate levels.
The CDC has released a series of updates on global prevention of FAPSBA, as more countries instituted fortification programs. In 2006 the decrease in FAPSBA through fortification programs around the world was estimated at 6.8%, in 2008 it was estimated at 9.1%, and in 2012 estimates ranged from 15.6% to 25.5%. The higher number of 25% is based on recent reports, suggesting that the threshold dose of folate necessary to prevent FAPSBA was lower than the originally proposed 0.4 mg/day. In addition, two recent case-control studies suggested that (1) full protection against FAPSBA was attained at levels lower than 0.4 mg/day, and (2) folate supplementation was no longer adding further protection against FAPSBA since the introduction of fortification. Together, these studies suggested that most of the FAPSBA prevention in the United States was through fortification and that the vast majority of FAPSBA around the world could be prevented with fortification programs alone.
A population-based study in Denmark investigated the effects of two public health measures on the incidence of myelomeningocele, specifically recommendations for folic acid supplementation and second trimester (18–19 week gestation) fetal ultrasound screening. The study concluded that recommendations for folate supplementation had no significant effect on the incidence of myelomeningocele because of lack of compliance. However, the second trimester prenatal ultrasound screening program was associated with a significant decrease in the rate of live-born babies with myelomeningocele. Data from a large study in China suggest that late administration of folate might have a protective role against neural tube defects. A strategy of high-dose (5 mg/day) folic acid rescue (to achieve rapid fetal levels) has been proposed for cases in which pregnancy is diagnosed before or in the provided time frame in women not taking folate supplementation.
The mechanism of the beneficial effect of folate is not established. One report raised the possibility that autoantibodies against folate receptors are present in as many as 75% of women who have had a pregnancy complicated by a neural tube defect. The autoantibody-mediated block of cellular folate uptake by folate receptors could be bypassed by administered folate, because the latter is reduced and methylated in vivo and is transported into cells by the reduced folate carrier. A related possibility is that the beneficial effect of folate could involve the metabolism of homocysteine to methionine , a reaction catalyzed by methionine synthase and necessitating a metabolite of folic acid (5-methyltetrahydrofolate). a
a References .
A critical enzyme in the synthesis of 5-methyltetrahydrofolate, methylenetetrahydrofolate reductase , is defective in 12% to 20% of cases of neural tube defects. One biochemical result of this disturbance is an elevation of homocysteine, which has been shown to produce neural tube defects in avian embryos. Another potential mechanism of a defect in homocysteine conversion to methionine is a disturbance in methylation reactions, for which methionine is crucial. Transmethylations of DNA, proteins, and lipids have far-reaching metabolic consequences.
Because some neural tube defects appear to be folate nonresponsive, other potential forms of nutritional supplementation have been explored. Inositol deficiency is the only known nutritional deficiency known to cause neural tube defects in mouse models. In addition, inositol blood levels are significantly lower in pregnant women carrying a fetus with a neural tube defect. Initial data from a randomized trial of periconceptional inositol supplementation in women with previous pregnancies complicated by neural tube defects are encouraging.
Management After Fetal Diagnosis.
The two-hit hypothesis for factors that determine the ultimate postnatal neurologic function of myelomeningocele survivors has significantly influenced fetal and delivery management of myelomeningocele. This hypothesis proposes that long-term neurologic function depends not only on the primary spinal defect but also on the accumulation of secondary, potentially treatable complications, including progressive injury to the exposed spinal neural tissue and the development of Chiari II lesions and hydrocephalus. In fact, several lines of evidence have implicated these secondary insults in the majority of the ultimate deficit. Specifically, earlier studies showed preservation of exposed neural elements and absence of both hindbrain herniation and hydrocephalus in autopsies of early but not later gestation fetuses with myelomeningoceles. Subsequent studies in midgestation fetuses showed loss of normal neural tissue at the level of the exposed tissue but not proximally. In addition to direct trauma, noxious substances in the amniotic fluid, including meconium, have been implicated. In myelomeningocele there is typically an accumulation of CSF in the subarachnoid space ventral to the cord at the level of the lesion (see Fig. 1.9 ); it has been suggested that the dorsal displacement of the cord exerts pressure on the dorsal cord neural elements by compression against the spinous defect. Clinically, these observations are supported by the fact that fetal leg movements are often noted earlier in gestation in areas where they are lost after birth. Other studies in rodent and ovine models suggested that secondary neural injury could be prevented or markedly attenuated and that normal hindbrain anatomy could be established after fetal closure of the spinal defect.
On the basis of these experimental data, initial nonrandomized clinical studies of intrauterine intervention were performed in human fetuses with myelomeningocele. These earlier studies reported a number of promising outcomes, including lower extremity function better than predicted from the anatomical level of the lesion, a significant decrease in hydrocephalus, and the need for postnatal shunt placement. Initial clinical reports also corroborated earlier experimental findings by showing reversal of hindbrain herniation as early as three weeks after human fetal myelomeningocele closure (possibly underlying the decreased need for shunt placement), as well as significantly fewer functional brain stem deficits ( Box 1.6 ). On the basis of these experimental and preliminary clinical results, a three-center randomized clinical trial of open prenatal versus conventional postnatal myelomeningocele repair was performed, in which the fetal surgery group with spinal lesions between the T1 and S1 levels underwent open surgical repair of the spinal lesion before 26 weeks’ gestation. The trial was terminated early for demonstrated benefit in the experimental fetal surgery group. Specifically, the fetal repair group required significantly fewer (40% vs. 82%) ventriculoperitoneal shunt procedures by 12 months of age, improved motor and mental composite scores at 30-month follow-up, and fewer (25% vs. 67%) moderate to severe Chiari II malformations ( Fig. 1.15 ). There was an overall improvement in the functional versus anatomical spinal level, as well as an increased incidence of ambulation without orthotic devices in the fetal surgery group. Specifically, 42% versus 21% of fetal surgery cases were walking independently at follow-up ( Box 1.6 ). However, the fetal repair group had a significantly higher rate of prematurity, chorionic membrane separation, and premature rupture of membranes, especially among those performed before 23 weeks’ gestation, and required more postnatal surgical untethering of the spinal cord. In addition, although no uterine ruptures occurred, 25% of women in this group had threatened or partial dehiscence of the hysterotomy site. Subsequent reports from the same center have corroborated those from the clinical trial but have shown that fewer than 30% of cases referred for evaluation actually underwent fetal myelomeningocele repair.
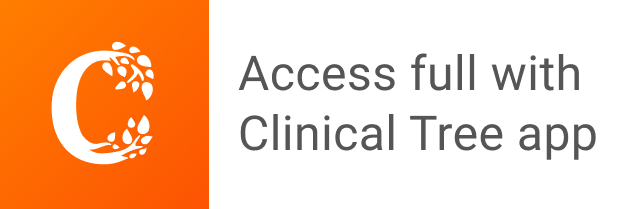