Fig. 8.1
Diurnal rhythms of sleep and waking states (top) and body temperature (bottom) in a squirrel monkey entrained to a 12/12-hr light/dark cycle. A awake; T transitional sleep; S non-REM sleep; R REM sleep. From Wexler and Moore-Ede [10], with permission

Fig. 8.2
a From bottom to top, plots of diurnal rhythms in core body temperature, brain temperature, sleep-waking states, and cortical EEG slow-wave activity (SWA) in a normal rat. b Plots of diurnal rhythms of the same variable in a, in a rat with bilateral lesions of the suprachiasmatic nucleus (SCN). From Baker et al. [16]
The sleep-related fall in CBT is an integrated thermoregulatory response, not a passive consequence of behavioral quiescence associated with sleep. The decline in CBT at the transition from waking to sleep persists in humans on a modified constant routine protocol (Fig. 8.3b), in which the level of behavioral activity during the 8-h prior to lights out is kept minimal and constant (Kräuchi et al. 2004) [2].


Fig. 8.3
a Schematic diagram of a human body indicating the small size of the heat producing core (shown in gray) relative to the large size of the heat loss regulating shell (shown in white) in a cool (20 °C) environment. During exposure to a warm ambient temperature (35 °C), the core expands significantly as blood flow to the periphery increase to promote facilitate heat loss to the environment. A similar expansion of the core relative to the shell occurs around the time of sleep onset and during the initial non-REM sleep episodes in humans, with the permission from Kräuchi [2]. b Dynamics of changes in CBT and Tsk during human sleep. Effects of an 8-h nocturnal sleep period (shaded area) on CBT, proximal and distal Tsk and DPG under constant routine conditions. Note the increases in distal Tsk and DPG that coincides with the steep decline in CBT early in the sleep period. The dashed line indicate a 8-h sleep deprivation period when the subjects usually sleep. Data from the 8-h sleep episode are double-plotted on the 8-h sleep deprivation period (gray dots). Modified from Kräuchi [2], with permission
At the transition from waking to sleep, the thermoregulatory system responds as if the thresholds for inhibition of heat production and for activation of heat loss responses have shifted to lower levels of CBT and/or Tsk. Wake-to-sleep transitions are accompanied by an integrated series of thermoregulatory adjustments, including a reduction in metabolic rate, increased heat loss to the environment through peripheral vasodilation (Fig. 8.3a, b) and, in warm environments, increased evaporative cooling. Body temperature falls to a lower regulated level which is maintained throughout the consolidated sleep period (Fig. 8.1). A simplified way of thinking about these changes is that the body’s thermostat has been reset to a lower level during sleep, i.e., a sleep-related lowering of an internal thermal “set point.”
With respect to thermoregulatory control, the body can be compartmentalized into a heat producing core and a heat loss regulating shell (Fig. 8.3a). The size of the shell can vary considerably depending on ambient thermal conditions. The shell is large in cool/cold environments when blood flow is diverted away from the limbs to the core to conserve heat (Fig. 8.3a). The shell contracts in warm/hot environment when blood flow to the limbs increases to promote heat loss. The thermoregulatory changes that occur at the wake-to-sleep transition redistribute heat from the core to the shell (Fig. 8.3a), increasing distal Tsk, facilitating heat loss to the environment, and augmenting the decrease in CBT (Fig. 8.3b).
The sleep-related reduction in metabolic heat production and consequent energy savings can be taken as evidence of an energy conservation function of sleep. The sustained lowering of CBT during the sleep episode also has a net energy conserving effect by reducing the CBT-to-ambient temperature gradient which reduces the rate of heat loss to the environment. This latter factor can be important in small mammals with a high surface to volume ratio that have inherently higher rates of environmental heat exchange. Behavioral aspects of sleep onset such as seeking a thermally comfortable nest and adoption of curled heat-conserving postures can also contribute to energy savings by minimizing heat exchange with the environment across an extended period of sleep.
Highly specialized energy conserving adaptations in mammals, such as hibernation and shallow torpor, can be viewed as extreme readjustments in thermoeffector thresholds [11]. Hibernation and torpor are characterized by prolonged and reductions in metabolic activity and body temperature. These events occur seasonally, when thermoregulatory demands are greatest and food availability is low. Bouts of hibernation and torpor are initiated as extensions of sleep episodes, and the dramatic fall in CBT is accompanied by integrated thermoregulatory adjustments similar to those observed during normal sleep [11]. Thermoregulation is not suspended during hibernation or torpor, but the thermoregulatory system behaves as if the thermal set point has been lowered, albeit dramatically so. This is most obvious in shallow torpor, where CBT is maintained well below normal sleeping levels, but significantly above ambient temperature.
Homeostatic responses to thermoregulatory challenges are modulated by arousal state. While CBT is maintained at reduced levels compared to waking during consolidated sleep episodes, thermoregulatory effector responses persist during non-REM sleep at normal or diminished levels, i.e., the magnitude of effector response to a thermal stimulus may be somewhat reduced compared to waking [12]. During non-REM sleep, there is a reduction in the thresholds and/or gains of autonomic heat and cold defense responses, with an effective expansion of the inter-threshold zone, which is the ambient temperature range between activation of heat production and heat loss responses.
Compared to non-REM sleep, thermoregulatory responses during REM sleep can be severely compromised [4]. This is particularly evident for cold defense responses, where the muscle atonia of REM sleep is incompatible with maintaining heat production via shivering or elevated muscle tone. Partial suppression of heat loss effector responses (e.g., panting) has also been described in REM sleep [13]. As a result of these thermoregulatory changes, animals are more vulnerable to thermal challenges during REM sleep compared to either waking or NREM sleep.
Exposure to hot or cold environments disrupt sleep (see below). This response may be adaptive in minimizing exposure to thermal stress during sleep when homeostatic compensation is weak. Thermal factors have undoubtedly played a role in the evolution of behavioral strategies for species-specific selection of sleeping environments, e.g., nests, sheltered locations, adoption of heat-conserving postures.
The seeming paradox of a sleep-related fall in CBT, combined with the selection of a relatively warm, insulated environment prior to sleep onset can be understood by considering the competing thermoregulatory requirements at work. Lowering metabolic rate and CBT during sleep can yield significant energy conservation benefits. However, diminished thermoregulatory adjustments to heat and cold challenge during sleep dictates that thermal homeostasis can be best achieved over the course of a several hour sleep period by selection of a sleeping environment that poses minimal thermal stress and ultimately maximizes energy conservation.
Interactions Between Circadian Rhythms in Sleep and Body Temperature
In healthy, entrained primates, including humans, habitual sleep onset occurs on the falling phase of the body temperature rhythm, 4–6 h before the CBT minimum (Fig. 8.1 and Fig. 8.3b). The onset of sleep is accompanied by an acceleration in the rate of the decline in CBT. In contrast, morning awakenings occur on the rising phase of the temperature rhythm, 1–3 h after the temperature minimum [2, 14]. The CBT rhythm is dictated by circadian rhythms in heat production and heat loss, with the rhythm in heat production phase advanced with respect to the heat loss rhythm [2]. A CBT rhythm persists during sleep deprivation or during constant routine protocols [15]. Nevertheless, behavioral changes associated with waking and sleep do exert positive and negative masking effects on the circadian temperature rhythms, and the magnitude of the 24 h peak-to-trough difference in body temperature is reduced during sleep deprivation or constant routine.
Circadian rhythms in body temperature and sleep are regulated by the biological clock located in the SCN of the hypothalamus. In experimental animals, lesions of the SCN abolish the 24 h rhythm in both sleep and body temperature [1]. In SCN-damaged rodents, circadian rhythms in sleep and body temperature are eliminated, yet daily total sleep amounts are unchanged. This indicates that the homeostatic regulation of sleep is largely undisturbed by SCN damage. However, the sleep-related fall in CBT persists in rodents with SCN lesions [16]. These animals exhibit ultradian rhythms in body temperature and sleep, and the two variables remain strongly coupled following SCN ablation, i.e., sleep episodes are reliably accompanied by falls in CBT (Fig. 8.2b).
The phase relationship between sleep and body temperature rhythms at sleep onset is an important determinant of subsequent sleep latency, sleep amount, and sleep quality. This has been most convincingly demonstrated in human subjects undergoing internal or forced desynchronization in environments without time cues. During internal desynchronization, sleep and body temperature rhythms free-run with different periods, and eventually, the two rhythms temporally dissociate [15, 17]. In forced desynchrony protocols, subjects adhere to a sleep-wake schedule significantly longer or shorter than 24 h (e.g., 28 or 20 h) and when this is done in the absence of time cues, body temperature and sleep rhythms dissociate as in internal desynchronization [15]. With both methods, over the course of several days the onset of the major sleep period will occur at all phases of the body temperature rhythm. Sleep episodes that are initiated on the falling phase of the temperature rhythm are associated with shorter sleep latencies, longer sleep episode durations, and maximal amounts of Stage N3 [15]. In contrast, sleep episodes initiated on the rising phase of the temperature rhythm are associated with longer sleep latencies, short sleep episode durations, more REM sleep but less Stage N3 sleep [15]. The functional importance of these phase relationships is demonstrated by the fact that, during internal and forced desynchronization, the phase of the CBT rhythm at sleep onset is a better predictor of sleep bout length than is prior time awake.
Collectively, findings indicate that thermoregulatory changes manifested at different phases of the CBT rhythm determine the expression of sleep. Activation of heat loss and suppression of heat production occurring during the falling phase of the temperature rhythm promotes sleep onset, sleep maintenance, and EEG slow-wave activity during sleep. Increased heat production and heat conservation accompanying the rising phase of the temperature promote waking.
Effects of Environmental and Body Temperature on Sleep
Human and animal studies demonstrate that the temperature of the sleeping environment is an important determinant of sleep amount and sleep quality. In all mammals studied, exposure to moderate hot or cold environments will suppress sleep and/or cause sleep fragmentation [4, 18]. In some species, REM sleep is more easily disrupted by thermal stress than non-REM sleep. In rats, where thermoregulatory responses during REM sleep are absent or weak, REM sleep suppression can occur under conditions of minimal thermal stress and in the absence of any significant change in non-REM sleep amounts [18, 19]. From a thermoregulatory perspective, the REM sleep suppressing effects of heat and cold exposure in these species are adaptive. Increased time spent awake or in non-REM sleep means that a lower threshold and stronger thermoregulatory responses will be evoked to maintain thermal homeostasis.
In contrast to the sleep suppressing effects of moderate-to-severe thermal stress, mild increases in ambient temperature or mild warming of the body can promote sleep. An animal’s thermoneutral zone is defined as the range of environmental temperatures at which resting metabolic rate is minimal and constant. Within the thermoneutral zone, minimal effort is required to maintain thermal homeostasis and thermal homeostasis is achieved largely through peripheral vasomotor adjustments that control heat exchange with the environment. Chronic exposure of rats to an environmental temperature that is at the upper end of the thermoneutral zone increases in daily total sleep time over baseline levels [20].
Whole-body warming, achieved by immersion in a hot bath a few hours prior to normal bedtime, promotes sleep in humans [5]. Hot baths prior to bedtime can reduce sleep latency and increase amounts of slow-wave sleep in the first half of the night. The timing of body warming is a critical factor. Optimum sleep-promoting effects of hot bath immersion are achieved 1–2 h prior to bedtime. Hot baths taken early in the day are ineffective. The critical variable in achieving sleep promotion appears to be that CBT remains slightly elevated above normal levels at bedtime, helping to promote a stronger heat loss response at sleep onset.
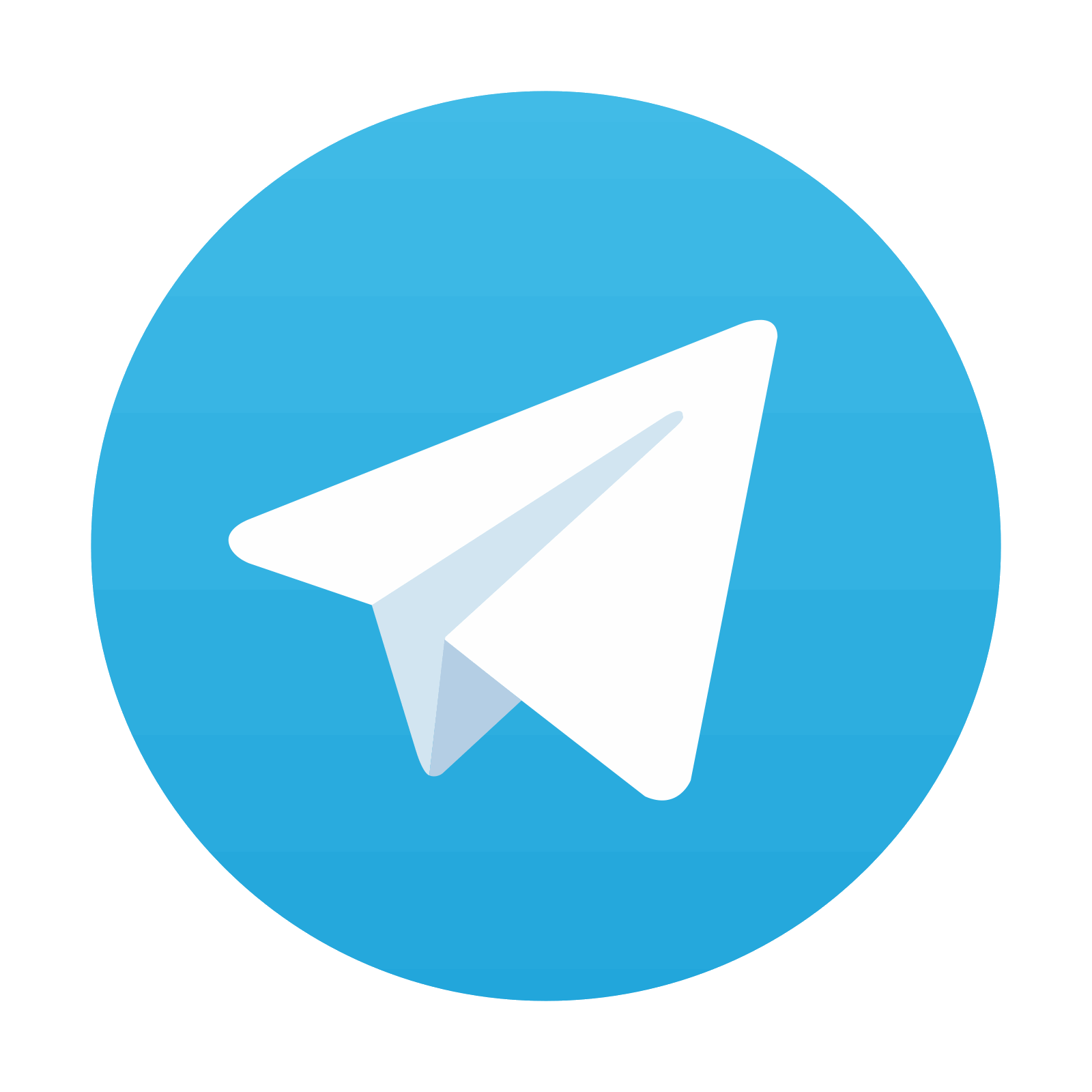
Stay updated, free articles. Join our Telegram channel
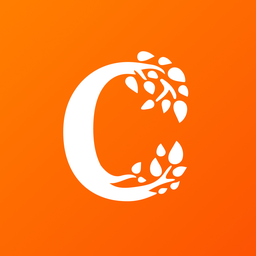
Full access? Get Clinical Tree
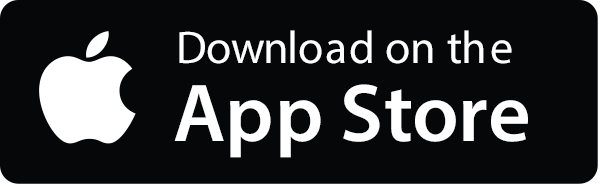
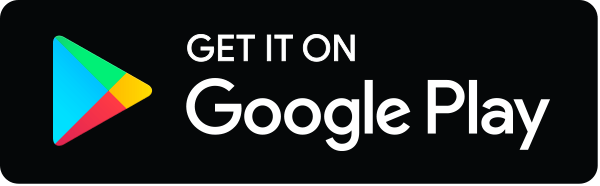