Fig. 5.1
Electroencephalographic (EEG) recordings of vigilance states (wake, NREM sleep, and REM sleep) in the rat and human. Wake in both species is characterized by cortical activation, of largely low-amplitude/fast-frequency activity. NREM sleep in the rodent is usually not differentiated between stages and exhibits large-amplitude delta (1–4 Hz) EEG activity. NREM sleep in humans is classified into 4 stages. In the first stage, the EEG frequency begins to slow, exhibiting alpha and theta activity. Both sleep spindles (7–14 Hz) and K-complexes are seen in NREM stage 2, as EEG amplitude increases and frequency further slows. In NREM sleep stage 4, also known as slow wave sleep, strong delta (1–4 Hz) activity of large amplitude is seen. During REM sleep, the EEG returns to a profile similar to wakefulness, with low-amplitude and high-frequency activity. In the rodent, the EEG exhibits strong theta (5–8 Hz) activity, most likely generated by the hippocampus. Human EEG recordings adapted [366]
Recent Neuroscience Technologies for Sleep Investigations
In recent years, new technologies in the field of neuroscience have been developed that are excelling our understanding of sleep and sleep-related disorders. The advent of optogenetic technologies now allows neurotransmitter-specific excitation and inhibition of neuronal populations [6, 7]. Light-activated ion channels (opsins) packaged in viruses (e.g., adeno-associated) are first intracerebrally injected in mice expressing Cre in specific populations of neurons. The neurotransmitter-select neuronal population of interest may then be excited or inhibited by means of light stimulation at the scale of millisecond precision. An alternative novel technique is the pharmacogenetic excitation or inhibition of select neuronal populations by means of Designer Receptors Exclusively Activated by Designer Drugs (DREADDs), in which cell type-specific expression of G-protein coupled receptors may be excited or inhibited by means of injection of select ligands such as inert clozapine-N-oxide [8, 9]. Cellular activation employing this technique, though, does not allow such precise temporal resolution as with optogenetics.
Gamma Band Oscillations (GBO)
Recent evidence points to the importance of GBO in the activated cortical EEG. During wake, the cortical EEG exhibits a predominance of low-amplitude beta (15–30 Hz) and gamma (30–120 Hz) cortical EEG activities. Neural signal transmission is enhanced as GBO occur, produced by the synchronized firing of cortical neuronal populations, providing a mechanism for coding information [10–12]. GBO abnormalities are symptomatic in various pathologies including schizophrenia, autism, and sleep disorders, and a strong association has been established between GBO and attention, perception, and memory [10, 13–15]. Moreover, GBO are enhanced after sleep deprivation largely in the prefrontal and frontal cortices of humans, suggesting an important sleep-related role of gamma rhythms [16].
Neural Mechanisms of GBO
Gamma rhythms are generated locally in the cortex by neural networks in which gamma-aminobutyric acid (GABA)ergic cortical interneurons containing the calcium binding protein parvalbumin (PV) interact with glutamatergic pyramidal cells (Fig. 5.2). Rhythmic fast-spiking inhibition of pyramidal cells produces GBO in the cortical EEG, and GBO frequency is set by the rate of depolarization and hyperpolarization of cortical PV and pyramidal neurons [17]. Electrophysiological investigations have shown that cortical PV neurons fire in phase with cortical EEG field oscillatory activity, especially GBO [18, 19]. GABA-A receptor antagonists applied to the hippocampal and cortical slice block GBO [20, 21]. Optogenetic excitation of cortical PV interneurons promoted GBO, and inhibition reduced GBO [13, 15, 22]. In magnetic resonance spectroscopy neuroimaging studies, cortical GABA levels increased during performance of a GBO-eliciting visual task [23, 24].


Fig. 5.2
Cortical circuitry generating gamma band oscillations (GBO). GBO are generated in the cortex by rhythmic fast-spiking GABAergic, parvalbumin (PV) cortical interneuronal inhibition of glutamatergic pyramidal cells. Returning glutamatergic projections excite PV GABAergic interneurons, enhancing oscillatory activity. Interneuronal–interneuronal mutual inhibitory connections between PV neurons, as well as electrical synapses and gap junctions, further promote synchrony (not illustrated)
Gamma band oscillations rhythmic activity is influenced by glutamatergic input from pyramidal cells back to GABA/PV neurons, as well as GABA/PV interneuronal–interneuronal interactions. Furthermore, synchronous PV rhythmic activity is promoted by gap junctions between cortical PV interneurons [25, 26]. In vitro investigations were able to elicit gamma oscillations in cortical slices, indicating that gamma oscillations are generated locally within the cortex [21, 27], and in vivo investigations also indicated that gamma oscillations are locally generated [28].
GBO During Wake
Many investigations indicate that synchronization of GBO between cortical subregions during wake provides a neural mechanism for intracortical communication [29–35]. The amplitude and synchrony of GBO correlated with the challenge/level of difficulty in a working memory task [36]. Recently, a strong correlation has been established between functional magnetic resonance imaging (fMRI) blood oxygen level-dependent (BOLD) signals and local GBO oscillatory activity [37, 38]. Although the temporal resolution of the BOLD signal is too slow to demonstrate GBO, these studies suggest that during select tasks that involve GBO, co-activation of cortical subregions occurs, possibly by means of direct anatomical connections, as well as synchronization by slower hippocampal and cortical oscillations, including theta and delta activity (also referred to as slow wave activity) [17].
Early Investigations of Wake-Regulating Neural Circuitry
During the 1920s, a worldwide epidemic of encephalitic lethargica led to many patients suffering from hypersomnia (i.e., excessive sleepiness). Von Economo analyzed the postmortem tissue of these patients and reported pathological damage in brain tissue located just posterior to the hypothalamus, at the junction of the mesencephalon and diencephalon [39]. A smaller subpopulation of encephalitic lethargica patients exhibited excessive wake, producing profound insomnia, and neurodegeneration was particularly noted in the hypothalamus. It was therefore concluded that an ascending system that involved subcortical projections to the cortex was involved in promotion of wake, and a sleep-inducing brain region resided in the hypothalamus. Later studies confirmed that these same brain regions play a role in the neural circuitry of the ascending reticular activating system (ARAS), reviewed below.
Frederick Bremer in the 1930s performed transection studies in the cat to identify brain regions that promote wakefulness [40, 41]. In one investigation, a particular transection/cut was made in the lower part of the medulla, termed encéphale isolé, which did not affect the cortical EEG profile. In another preparation, called cerveau isolé, a transection was made at the intercollicular region of the midbrain, rendering the animal in a sleep-like state. These findings suggest that the transition to and maintenance of sleep involve a disconnection of subcortical and cortical brain regions.
Moruzzi and Magoun demonstrated that stimulation of the brainstem reticular formation was very effective in eliciting a cortical EEG profile indicative of wake [42]. The concept of the ascending reticular activating system (ARAS) (Fig. 5.3) was then developed, describing the ascending circuitry originating in the reticular formation that is responsible for wakefulness [43–45]. The ARAS includes a dorsal and ventral pathway, both of which originate in the reticular formation, particularly the rostral pontis oralis (RPO). The dorsal pathway involves reticular formation input to thalamic nuclei that, in turn, strongly project to much of the cortex. Most of the ascending projections for this pathway are excitatory/glutamatergic, including reticular formation input to the thalamus and thalamocortical projections. The ventral pathway again involves projections originating in the reticular formation, now projecting to regions in the hypothalamus, namely the basal forebrain. The basal forebrain in turn sends direct connections to the cortex, again promoting wake. A number of studies have described strong input from the basal forebrain to widespread regions of the cortex, including cholinergic, GABAergic, and, to a lesser extent, glutamatergic basal forebrain input [43–45]. In addition, the medial septum/vertical limb of the diagonal band of Broca of the basal forebrain sends GABAergic and cholinergic input to the hippocampus. GABAergic input paces theta frequency oscillatory activity, seen during wake-related movement and select memory processing, as well as throughout REM sleep [46–48]. The ventral pathway involves glutamatergic output from the reticular formation, as well as basal forebrain glutamatergic projections terminating on the cortex. In addition, a multitude of other neurotransmitters, including acetylcholine, serotonin, noradrenaline, and the neuropeptide orexin, are involved.


Fig. 5.3
Ascending reticular activating system (ARAS) promotes wakefulness. The dorsal pathway (dashed) originates in the reticular formation and neighboring dorsal raphe (DR), locus coeruleus (LC), parabrachial (PB), and laterodorsal (LDT) and pedunculopontine (PPT) tegmental nuclei. These regions project to thalamic nuclei that in turn send widespread projections to the cortex. The ventral pathway (solid) also originates in these same pontine and midbrain nuclei, which project directly to the basal forebrain (BF) of the hypothalamus, as well as indirectly through the lateral hypothalamic (LH) and tuberomammillary (TMN) nuclei. BF in turn projects diffusively to the cortex, promoting wakefulness. POA preoptic area; SN substantia nigra; VTA ventral tegmental area
Wake-Promoting Neurotransmitters
Acetylcholine
The excitatory neurotransmitter acetylcholine is localized in two essential brain nuclei significantly involved in wake promotion. The first brain region is located in the midbrain tegmentum, including the laterodorsal tegmental (LDT) and pedunculopontine (PPT) tegmental nuclei. The second region is the basal forebrain and includes the medial septum/vertical limb of the diagonal band of Broca, horizontal limb of the diagonal band, magnocellular preoptic nucleus, substantia innominata, and nucleus basalis. Neurochemical measurement revealed increased levels of acetylcholine in the thalamus and cortex during both wake and REM sleep [49–52].
Electrophysiological recordings have revealed a significant association of basal forebrain neuronal activity with cortical activation [44, 45]. Investigations employing post hoc juxtacellular labeling and single unit recording in the head-restrained rat demonstrated that basal forebrain cholinergic neuronal activity was significantly elevated during wake and REM sleep, when compared to NREM sleep [53–56]. Due to optogenetic excitation of basal forebrain cholinergic neurons, there was an increase in transitions from NREM sleep to wake or REM sleep, overall amounts of wake increased, and stimulation during wake and REM sleep sustained cortical activation [57, 58], although recent optogenetic studies indicate that the wake-promoting effect of basal forebrain cholinergic neurons is less potent than that of basal forebrain GABAergic PV populations (see below).
Labeling of c-Fos, an immediate early gene, and its related protein in the brain is a useful tool to document neuronal activation associated with calcium influx [59, 60]. c-Fos activation was documented in basal forebrain cholinergic neurons immediately after the animal was sleep deprived, indicating that these neurons were indeed activated by sleep deprivation [61–63]. Furthermore, the amount of c-Fos activation correlated with the amount of wake observed in the preceding hour before sacrifice [61, 62]. Specific lesions of basal forebrain cholinergic neurons employing the neurotoxin saporin decreased wakefulness and cortical activation [45, 64–68]. Although various wake EEG indices were affected following basal forebrain lesions, wake state total amounts remained intact. Presumed GABAergic and glutamatergic cell populations in the basal forebrain remain following cholinergic-specific lesioning, suggesting that non-cholinergic basal forebrain neurons may also play a role in promotion of wake. Furthermore, alternative ascending systems that do not involve the basal forebrain also promote wake, such as the dorsal ARAS pathway.
LDT/PPT cholinergic neurons send extensive projections to the basal forebrain, thalamus, and cortex, providing multiple routes to influence cortical activation. Numerous electrophysiological studies have shown tegmental neuronal firing in relation to wake and REM sleep [45, 69, 70]. In a recent study employing juxtacellular labeling and single unit recordings, identified cholinergic LDT/PPT neurons exhibited increased firing in relation to cortical EEG during wake and REM sleep [71].
Serotonin
Most of all nuclei in the ARAS receive a serotonergic innervation originating in select brainstem/midbrain raphe nuclei, particularly the dorsal and median raphe [72–75]. Due to the variety of serotonergic receptors (14 subtypes at last count), the role of serotonin in the ARAS is quite complicated. Early work by Jouvet and colleagues reported that depletion of serotonin by means of p-chlorophenylalanine (PCPA) produced insomnia, leading to the initial proposal that serotonin is sleep promoting [76, 77]. Later investigations revealed that such serotonergic depletion also influenced body temperature and movement, hence promoting insomnia. Still later investigations concluded that serotonin acts to promote wake and inhibit REM sleep (our current understanding). Brainstem dorsal raphe neurons are most active during wake, significantly decrease their activity during NREM sleep, and largely cease activity during REM sleep [78, 79]. Notably, juxtacellular labeling and single unit recordings revealed that most dorsal raphe neurons fire during wake, yet a smaller population were sleep active [80, 81], demonstrating the complex heterogeneity of serotonergic cell populations. Optogenetic cell-specific activation of dorsal raphe serotonergic neurons promoted wakefulness, at the expense of NREM sleep amounts [82].
Numerous pharmacological and genetic knockout investigations have also suggested a role of serotonin in wake promotion. Neurochemical measurement revealed that serotonin levels were highest during wake in the dorsal raphe, as well as many of its efferent targets including the anterior hypothalamus, basal forebrain/preoptic region, hippocampus, and frontal cortex [83–87]. Nevertheless, some studies were unable to demonstrate changes in serotonergic levels in brain regions including the cortex and hippocampus during sleep deprivation, again illustrating the complexity of the serotonin system in sleep regulation [87, 88].
Infusion of 8-hydroxy-2-dipropylaminotetralin hydrobomide (8-OH-DPAT), a largely selective 5-hydroxytryptamine (5HT)-1A receptor agonist, into the dorsal raphe nucleus promoted REM sleep, by means of activation of the 5HT-1A autoreceptor on these serotonergic neurons [89, 90]. Similar to these findings, 8-OH-DPAT activation of the 5HT-1A autoreceptor in the median raphe nucleus promoted hippocampal theta activity [91, 92], providing a mechanism by which the serotonergic median raphe may suppress theta activity indicative of REM sleep. Neonatal treatment of mice with the selective serotonin reuptake inhibitor escitalopram induced depression and anxiety-like behavior, as well as increased REM sleep [93]. The serotonin transporter knockout mouse expressed depression-like behavior, and levels of both REM sleep and serotonin were elevated [94–97]. Although these findings seem to contradict the proposed role of serotonin as a wake-promoting neurotransmitter, REM sleep promotion in the knockout mouse may be due to a down regulation of 5HT-1A receptors on cholinergic neurons in response to the increase in serotonergic tone. Neonatal introduction of compounds that block the 5HT-1A receptor in the serotonin knockout mouse reversed both behavioral and cortical abnormalities, including REM sleep stabilization [98].
Noradrenaline
Noradrenergic brain regions, particularly the brainstem locus coeruleus (LC), also play a major role in promoting wakefulness. The LC sends projections to a number of ARAS-related nuclei, including the basal forebrain, midline thalamus, and cortex [72, 73, 99–101]. Noradrenaline may act as an excitatory or inhibitory neurotransmitter, depending on the type of postsynaptic noradrenergic receptor. The majority of ARAS-related noradrenergic projections are excitatory, acting on α1 receptors [45, 102–105]. Noradrenergic projections to the sleep-related preoptic hypothalamic region, some cholinergic tegmental neurons, and the LC itself act on α2 receptors [106, 107]. LC neurons are most active during wakefulness, and largely cease activity during NREM and REM sleep [108–110]. Non-specific lesions of LC do not drastically alter vigilance states, though [111–115], suggesting that the LC may not be sufficient to alone produce wake, but still influences cortical activation. Recent studies suggest that stress-induced noradrenergic activation may contribute to insomnia, further suggesting that noradrenaline acts to promote wakefulness [116, 117]. Recently, cell-specific optogenetic excitation of noradrenergic LC neurons promoted NREM sleep to wake transitions, overall amounts of wakefulness, and locomotion [118]. Furthermore, optogenetic inhibition of these same neurons decreased amounts of wake and promoted the slow wave activity indicative of NREM sleep. Pharmacogenetic excitation of noradrenergic LC neurons by means of Designer Receptors Exclusively Activated by Designer Drugs (DREADDs) produced cortical activation during isoflurane general anesthesia, as slow wave activity was reduced [119], again implicating noradrenergic systems as a major player in arousal.
Dopamine
Dopaminergic neurons, located in specific brain nuclei including the substantia nigra (SN) and ventral tegmental area (VTA), project to the basal forebrain and the cortex, and returning projections from a number of ARAS-related nuclei have been described [120–122]. Midbrain dopaminergic neuronal burst firing is associated with locomotion, select cognitive functioning, and reward [123–127]. Although single unit recordings were not able to directly correlate SN or VTA unit firing with changes in vigilance state [128], dopaminergic neuronal activity fluctuated across vigilance states, as burst firing increased in frequency during wake [122, 129–131]. Another population of dopaminergic neurons in the ventral periaqueductal gray expressed c-Fos immediately following sleep deprivation, indicating neuronal activation during wake, and these activated neurons were found to project to a number of forebrain ARAS-related nuclei [132]. Of note, treatment of narcolepsy with dopamine-stimulating compounds has proven somewhat successful, allowing stabilization of the vigilance state profile [133, 134].
Histamine
Histaminergic neurons located in the bilateral tuberomammillary nuclei of the hypothalamus (TMN) also play a role in promotion of wake. Notably, first-generation antihistamines have a sedating effect, acting as antagonists on H1 receptors located on a number of wake-promoting nuclei [135]. Histaminergic TMN neurons project to a number of ARAS-related nuclei, including direct projections to the cortex [136–139]. Histaminergic neurons demonstrate a discharge profile similar to noradrenergic neurons, highly increased activity during wake and significantly decreased activity during sleep [140–142]. Levels of histamine in the posterior hypothalamus were highest during wake and decreased during both sleep states [143]. Both non-specific and histamine-specific lesions of TMN, as well as chemical manipulations, suggest that TMN histamine neurons are indeed wake promoting [141, 144]. Additionally, brain mast cells, which produce approximately 50% of brain histamine, are also implicated in promoting wakefulness [145].
Orexin
Recent investigations within the last 2 decades have described a specific population of neurons that express the neuropeptide orexin (also called hypocretin) in the perifornical (near fornix) region of the posterior hypothalamus. Neuroanatomical investigations revealed strong orexinergic input to ARAS wake-promoting nuclei [146, 147]. Orexinergic neurons fire most during wake, decrease activity during NREM sleep, and are largely silent during REM sleep, except for activation during occasional muscle twitching [148]. Levels of orexin are highest during wake in the lateral hypothalamus [149], and c-Fos studies revealed activation of orexinergic LH neurons immediately following sleep deprivation [150].
Optogenetic cell-specific stimulation of orexinergic neurons produced an increase in NREM–wake and REM–wake transitions [151]. Furthermore, these neurons preferentially fired at frequencies of 5–30 Hz, resembling the firing pattern recorded in orexinergic neurons during cortical activation. Optogenetic stimulation of noradrenergic LC neurons produced NREM sleep to wake transitions of a very short latency (~5 s) compared to orexinergic stimulation (~10–30 s), leading to the proposal that LC may be a primary effector of arousal [152]. Pharmacogenetic excitation of orexinergic neurons by means of DREADDs produced neuronal activation, reflected by c-Fos protein labeling, as well as increased time in wake at the expense of both NREM and REM sleep amounts [153]. Also, inhibition of orexinergic neurons with DREADDs decreased wake and elevated total amounts of NREM sleep.
Symptoms of the sleep disorder narcolepsy include hypersomnia, hallucinations, cataplexy, sleep-onset REM sleep, and sleep paralysis. Investigations in the narcoleptic canine model described a deficit in the orexin type 2 receptor [154]. Also, wake was significantly decreased in orexin knockout mice in which the orexin precursor prepro-orexin was deleted [155]. In these animals, the number of transitions between vigilance states was significantly increased, similar to that seen in narcoleptic patients [155]. Orexin-specific lesioning and genetic disruption studies produced a number of symptoms in animals similar to that seen in the narcoleptic, including increased state transitions and intrusion of REM sleep-like characteristics into wake [156–159]. Analysis of postmortem brain tissue collected from narcoleptics demonstrated a significant loss of orexin neurons [160, 161], and low cerebrospinal fluid levels of orexin were reported in cataplectic and narcoleptic patients [162, 163]. Recent investigations have attempted to reverse the neurodegeneration of orexin neurons in LH by means of systemic administration, gene transfer, and cell transplantation of orexin [164–169]. Furthermore, treatment of human narcoleptics with orexin improves a number of symptoms, including sleep abnormalities [169, 170].
Investigations in the narcoleptic canine model suggest that noradrenergic and serotonergic cell populations may play more of a role in the maintenance of muscle tone during wake, and histamine in maintenance of cortical activation. Cataplexy, such as seen during narcolepsy, involves a sudden loss of muscle tone, although consciousness remains. During cataplectic attacks in the dog, noradrenergic and serotonergic cell populations largely ceased firing, but histaminergic neurons remained active [142]. Histamine may therefore play a unique role in promoting wake, particularly cortical activation. Abnormalities in histamine levels in the CSF of narcoleptic patients have been reported [171, 172]. Notably, postmortem analysis of human narcoleptic tissue revealed a significant increase in the number of TMN histamine neurons [173].
Treatment of narcolepsy includes stimulant-like compounds, including dopamine reuptake inhibitors, which improve the sleep profile of the narcoleptic patient, and adrenergic, dopaminergic, and serotonergic reuptake inhibitors are effective in the treatment of cataplexy symptoms [45, 133, 134, 174]. Sodium oxybate has been shown to improve the sleep/wake profile and some cataplectic symptoms in narcoleptic patients [175–177]. Also, orexinergic antagonists are being pursued as a treatment for insomnia [178–180].
Gamma-Aminobutyric Acid (GABA) and Glutamate
In addition to sleep promotion, GABAergic neurons in the cortex and select subcortical regions, such as the basal forebrain, also play a role in the promotion of wake. GABAergic PV neurons play a necessary role in the generation and maintenance of cortical GBO, by means of interaction with cortical pyramidal neurons (reviewed above). Select basal forebrain cortically projecting GABA neurons have been shown to fire in relation to cortical GBO activity [56], and caudal basal forebrain lesions with ibotenic acid, presumably targeting GABAergic neurons, reduced cortical activation and GBO [65]. A number of wake-promoting neurotransmitters, including those reviewed here, excite basal forebrain GABA neurons, which in turn may activate arousal systems that generate both cortical GBO and hippocampal theta rhythms [56]. Optogenetic excitation of basal forebrain GABAergic/PV neurons entrained GBO in the mouse [181]. In particular, 40 Hz optical stimulation of basal forebrain PV neurons increased cortical GBO. Thus, the basal forebrain and particularly its PV neuronal projecting population play a role in both promoting cortical GBO, as well as fine tuning the pattern of cortical activity during wakefulness.
As previously mentioned, both the dorsal and ventral pathways of the ARAS include glutamatergic excitatory projections. The dorsal pathway includes glutamatergic input from the reticular formation to the thalamus, as well as glutamatergic thalamocortical projections. The ventral pathway of the ARAS includes glutamatergic input from the parabrachial nucleus and reticular formation to the basal forebrain, and basal forebrain projections to the cortex include cholinergic, GABAergic, and, to a lesser extent, glutamatergic input.
Subcortical Modulation of GBO
Although GBO are generated locally in the cortex, subcortical input to the cortex influences GBO, beyond basal forebrain PV influence. Electrical stimulation of the brainstem reticular formation, and its presumed glutamatergic input to the hypothalamus and thalamus, promoted GBO, demonstrating that central activation of ARAS provides a mechanism of synchronization of different cortical subregions [182, 183]. Cholinergic brain nuclei of the ARAS promote high-frequency cortical oscillatory activity including GBO during wake and REM sleep [184–189]. Electrical stimulation of cholinergic brainstem tegmental neurons enhanced thalamocortical cell firing at gamma frequency, as well as the cortical GBO itself [190]. Lesions of basal forebrain cholinergic neurons attenuated high-frequency EEG activity, including GBO, particularly when lesions included the caudal basal forebrain cholinergic cell population [65, 68, 191–194]. Serotonin acts to inhibit cholinergic neurons in both the basal forebrain and tegmental zones [187, 195, 196], attenuating high-frequency beta and gamma activities [188].
NREM Sleep
EEG Profile of NREM Sleep
The cortical EEG profile of NREM sleep includes higher-voltage (amplitude) field oscillations, compared to wake, as well as a slowing of oscillatory activity frequency (Fig. 5.1). Human NREM sleep has been traditionally scored as four stages based on the Rechtschaffen and Kales (R and K) criteria [197]. More recently, the American Academy of Sleep Medicine suggested that NREM sleep may be classified in three stages [198]. N1, NREM sleep stage 1, includes a predominance of theta (4 < 8 Hz) and alpha (8–13 Hz) activity. N2, NREM sleep stage 2, includes both sleep spindles (described below) and K-complexes. K-complexes are brief high-voltage spikes of around 110 µV, and last approximately 0.5 s. They are unique to NREM stage 2 and are a combination of neocortical slow oscillations and spindles [44]. N3 includes that previously described by R and K criteria as stages 3 and 4. This stage is “deeper” NREM sleep, characterized by a predominance of slow delta frequency (1 < 4 Hz) oscillations, and therefore also called “slow wave activity” (SWA). Furthermore, throughout NREM sleep a neocortical slow oscillation (0.5–1 Hz) occurs, which plays a role in thalamic and intracortical synchronization, particularly synchronizing sleep spindles [44, 199–201]. During NREM sleep, EMG recordings exhibit lower muscle tone and little movement, and eye movement is usually absent. In rodent studies, stages of NREM sleep are usually not differentiated.
Sleep Spindles
Sleep spindles are most prominent in human NREM stage 2 [44, 45]. These spindles in humans are of the frequency of 11–16 Hz, lasting around 1 s, and are generated by interplay between the thalamic reticular nucleus (TRN) and thalamocortical neurons. Cortical EEG recordings in the mouse revealed an increase in cortical oscillatory activity of ~11 Hz during early NREM sleep, and also prominent during NREM–REM sleep transitions [202–204]. Sleep spindles promote the deafferentation of thalamocortical input to the cortex that is seen during NREM sleep, and GABAergic TRN neuronal activity particularly paces these oscillations [205, 206]. Optogenetic excitation of TRN GABAergic neurons induced burst firing in thalamocortical neurons, as well as produced cortical spindle activity [204] and increased the duration of NREM sleep [207]. LDT/PPT cholinergic input acts, in part, to inhibit TRN activity, thereby desynchronizing the TRN-thalamocortical oscillatory network and promoting wake and cortical activation [45, 206]. Moreover, basal forebrain neurons project to the TRN, particularly its rostral aspect [208], and chemical lesions in the basal forebrain attenuated spindle activity [209].
Sleep spindles are of current interest since they are markedly reduced in schizophrenia [210, 211]. Therefore, spindle activity is probably the most reliable biological marker for this disorder in sleep. Treatment with eszopiclone, a hypnotic with GABA-α2 receptor agonistic activity, lessened the sleep spindle deficit seen in schizophrenics, as well as improved memory consolidation [212]. During NREM sleep, cortical slow wave activity coordinates the timing of hippocampal ripples as well as prefrontal spindle activity. In a rat model of schizophrenia, fragmented NREM sleep and impaired slow wave propagation was noted, as well as attenuated prefrontal spindle activity, suggesting that NREM sleep abnormalities such as seen in schizophrenia may produce a decoupling of hippocampal and neocortical activity [213].
Delta Slow Wave Activity (1 < 4 Hz)
As previously mentioned, NREM deep stage activity is indicated by cortical EEG oscillations predominantly in the delta frequency of 1 < 4 Hz, referred to as slow wave activity (SWA). Delta activity occurs as thalamocortical neurons are hyperpolarized. Phasic input to these hyperpolarized thalamocortical neurons produces a low-threshold calcium spike, on which sodium-mediated spikes occur (low-threshold burst). Another subsequent hyperpolarization then occurs, generating the delta frequency oscillation. Blocking thalamic input to the neocortex decreased sleep spindle activity and the frequency of NREM sleep SWA [214]. SWA is also blocked by cholinergic, serotonergic, and noradrenergic excitatory input to either thalamocortical or cortical neurons [45]. Recent investigations indicate that SWA originates locally in individual cortices during sleep [201, 215–217]. For example, individual cortical regions exhibit SWA during sleep deprivation/forced wakefulness, although other cortical subregions exhibited cortical EEG activity indicative of wake [216]. As sleep deprivation progressed, more cortical subregions exhibited sleep-like SWA, indicating an increased homeostatic response to extended wakefulness.
GBO During NREM Sleep
Slow oscillations seen during NREM sleep, including the slow 0.5 Hz wave and delta activity, may underlie limited GBO activity in NREM, activity that is much less than in wakefulness and REM sleep. Recent investigations have investigated high-frequency changes in the human cortical EEG during both NREM and REM sleep [218–221]. The up state of SWA is caused, in part, by prolonged cortical pyramidal depolarizations, and associated GBO were recorded in association with these up states, localized largely in the frontal cortex [200, 218, 219, 222]. This up state is followed by a down state, produced by extended hyperpolarization of pyramidal neurons due to GABAergic interneuronal inhibition. In one investigation, GBO were reported to be associated with this down state, specifically seen in temporal cortical regions [218].
NREM Sleep-Active Neurotransmitters: GABA and Galanin
Sleep-active GABAergic neuronal subpopulations are located in the preoptic nuclei of the hypothalamus. Lesioning and electrophysiological studies suggest that a proportion of neurons in the basal forebrain and the neighboring preoptic region promote NREM sleep [45, 223, 224]. c-Fos protein was expressed in preoptic GABAergic neurons, including the ventrolateral (VLPO) and median preoptic (MnPN) nuclei, during natural sleep and after recovery from sleep deprivation, when sleep was particularly enhanced [225, 226]. The VLPO is uniquely positioned to affect ARAS-related nuclei, for it receives input from a number of excitatory wake-related nuclei [227], and reciprocally projects to many of these same nuclei using GABA and galanin as inhibitory neurotransmitters [228]. Specific lesions to the core of VLPO produce largely NREM sleep disturbances [229]. Select basal forebrain/preoptic neurons increased firing during NREM sleep, and juxtacellular labeling and single unit recording techniques revealed that many of these neurons were GABAergic [54]. MnPN neurons fired immediately preceding entrance into NREM sleep, and continued throughout NREM sleep, and VLPO neurons were active largely during NREM sleep [223, 224, 230].
Evidence indicates that cortical neuronal nitric oxide synthase (nNOS) neurons modulate SWA. nNOS knockout mice exhibited disrupted NREM sleep and decreased amounts of SWA after sleep deprivation [231]. In addition, mice injected centrally with the nNOS antagonist 3-bromo-7-nitroindazole have reduced SWA compared to vehicle injections [232]. Recently, cortical GABAergic neurons that co-express nNOS and the neurokinin 1 receptor (i.e., the primary receptor for the pro-inflammatory molecule substance P) were activated during recovery sleep after sleep deprivation, assessed with c-Fos immunoreactivity [231, 233–236]. The activation of these cortical neurons is associated with changes in SWA and likely play a role in regulating SWA.
As mentioned, the VLPO is reciprocally connected with a number of ARAS-related nuclei [237]. Saper and colleagues proposed that this circuitry was reminiscent of a “flip-flop” switch as described in electrical engineering [238], depicted in Fig. 5.4. One exception of this mutual inhibition between wake and sleep-active nuclei is that histamine does not directly affect VLPO activity [239]. TMN histamine may inhibit VLPO indirectly, though, considering TMN histamine neurons are largely GABAergic. As shown in Fig. 5.4, orexin acts to switch the “flip-flop” between wake and NREM sleep states. Abnormalities in orexinergic circuitry would lead to abnormal vigilance state regulation, which is similar to that seen in narcolepsy. Of note, orexin knockout mice exhibit excessive vigilance state changes [158]. Orexin therefore is an essential player in vigilance state regulation, promoting transitions between wake and sleep in the “flip-flop” model. Recent optogenetic investigations suggest that LC noradrenergic neurons may be a primary effector of arousal, transferring orexinergic LH activity [152].


Fig. 5.4
“Flip-flop” switch model of sleep/wake transitions [238]. Histaminergic neurons of the tuberomammillary nucleus (TMN), serotonergic neurons of the dorsal raphe nucleus (DR), and noradrenergic neurons of the locus coeruleus (LC) promote wakefulness and inhibit ventrolateral preoptic (VLPO) sleep-active neurons. Lateral hypothalamus (LH) orexinergic neurons excite wake-active neurons, stabilizing the wake state. During sleep, GABAergic/galaninergic VLPO GABAergic/galaninergic neurons inhibit the wake-promoting nuclei, including LH. Mutual inhibition between these wake- and sleep-promoting nuclei produces vigilance state transitions
The Circadian Drive to Sleep
The two-process model of sleep regulation was first proposed by Borbély and colleagues [240], involving both a homeostatic (process S, reviewed later) and circadian (process C) component. Circadian rhythms of behavior follow an approximate 24-h periodicity, and both endogenous internal and exogenous environmental oscillators influence vigilance state regulation. Such environmental cues are termed “zeitgebers” and include light and food. The interactions of both process S and C produce a distinct pattern of daily wakefulness and nocturnal sleep in humans [241–243]. Overviews of circadian rhythmicity and its influence on behavior are beyond the scope of this chapter and available elsewhere [242–244].
A major internal oscillator of circadian rhythmicity is the suprachiasmatic nucleus of the hypothalamus (SCN). The SCN is the primary internal biological clock that influences sleep/wake regulation [243–245]. c-Fos neuronal activation studies and electrophysiological recordings indicate that SCN activity is highest during the inactive period of animals [243, 246]. Lesion studies of SCN produced disrupted rhythmicity of behavior, including vigilance states, as well as hormonal secretions. Notably, although vigilance state regulation was disrupted in SCN-lesioned animals, total amounts of sleep/wake over 24 h remained unchanged.
NREM Sleep Homeostasis
As posited in the two-process model of sleep regulation, sleep is influenced by both process S, the homeostatic drive to sleep based on SWA, and process C, the circadian drive to sleep [240]. Process S is an increase in the propensity to sleep due to the previous time spent awake. That is, the longer an animal (or person) stays awake, neural mechanisms act to increase the drive for sleep. Numerous studies have demonstrated that, as wake is extended with techniques such as acute sleep deprivation/fragmentation, the homeostat is pushed, and the intensity of NREM sleep, measured as SWA, is significantly increased, evident in the recovery period following sleep disturbance. Recent investigations suggest that SWA and sleep are regulated independently [247]. Some experimental sleep disruption protocols model the sleep restriction experienced by many individuals, in which only short amounts of sleep per day are allowed. SWA in the recovery period following multiple days of sleep restriction did not lead to enhancement of SWA in the rat [233, 248–250], yet functional detriments such as impaired cognition persisted [251]. Therefore, chronic sleep loss conditions appear to exhibit a compensatory allostatic response not applicable to the two-process model [249, 250].
Metabolic Homeostasis
Adenosine Triphosphate. One proposed hypothesis of sleep regulation is that sleep allows the replenishment of energy stores diminished during wake [252, 253]. Evidence indicates that adenosine triphosphate (ATP) is released into the extracellular space with increased waking activity and functions to enhance sleep [254]. The purine adenosine is the product of ATP degradation by enzymes including 5′-ectonucleotidase, which occurs as energy stores are used in cellular metabolism [255, 256]. Mice lacking 5′-ectonucleotidase have an attenuated NREM sleep response to sleep deprivation [257]. ATP levels were shown to increase in the first few hours of NREM sleep, further supporting the relevance of the energy hypothesis; moreover, the increased ATP was associated with a reduction in phosphorylated adenosine monophosphate kinase and hence a favoring of anabolic processes [258].
Adenosine. Adenosine acts as a somnogenic neurotransmitter, promoting sleep by means of interaction with adenosine-specific receptors located in various brain regions [255, 256, 259, 260]. As an organism stays awake, adenosine levels increase, acting on adenosine receptors to inhibit wake-active nuclei of the ARAS, namely the basal forebrain and the cortex. Levels of extracellular adenosine increased selectively in the basal forebrain and the cortex as the sleep homeostat is pushed by means of either sleep deprivation or fragmentation [61, 261–264]. Adenosine diurnally fluctuates, as level rise during the dark (active) period in the rodent, and decrease as the light (inactive) period progresses [263–265]. In epileptic human subjects, indwelling microdialysis sampling in the cortex, hippocampus, and amygdala demonstrated a diurnal fluctuation, as extracellular adenosine levels were elevated during the active day [266].
Administration of adenosine or adenosine agonists into the basal forebrain diminished wake and promoted sleep, and adenosine antagonists increased amounts of wake [255, 256]. Of note, both caffeine and theophylline are adenosine receptor antagonists, explaining the wake-promoting effect of these compounds. Adenosine acts as a somnogen by means of adenosine 1 receptor activation in the basal forebrain [45, 255, 256, 262]. Mice lacking the adenosine 1 receptor gene have a decreased SWA response during the sleep recovery after sleep deprivation, as well as cognitive dysfunction in a working memory task, suggesting that adenosine 1 receptor activation is necessary for sleep homeostasis [267]. Both astrocytes and neurons of the basal forebrain release adenosine and modulate the homeostatic drive for sleep [45, 255, 268]. Recently, NREM sleep has been shown to play a role in metabolic homeostasis by means of a “glymphatic” mechanism [269]. In this study, as natural spontaneous sleep progressed, interstitial space expanded, allowing an increased exchange of cerebrospinal and interstitial fluids. The travel clearance of administered beta amyloid was increased during sleep, suggesting that sleep serves the purpose of removing toxic waste products that accumulate in the brain during wake.
Adenosine also acts as a somnogen in the subarachnoid space just ventral to the basal forebrain and the neighboring preoptic area, including the VLPO, largely by means of adenosine 2A receptor activation that disinhibits VLPO GABAergic/galaninergic sleep-promoting neurons [270]. Prostaglandin 2, another proposed somnogen, was intracerebrally injected into this region, and c-Fos neuronal activation was evident in VLPO neurons [271]. Additionally, NREM sleep amounts were significantly increased after prostaglandin VLPO injections [272], which promoted adenosine release [273].
Nitric oxide. Nitric oxide (NO) production promotes adenosine release during wake, such as seen during sleep deprivation [274, 275]. In vitro administration of NO or NO donors increased adenosine production in the basal forebrain and hippocampus [276, 277], and in vivo intracerebral injection of NO donors into the basal forebrain increased NREM sleep and basal forebrain adenosine release [278]. Therefore, as the sleep homeostat is elevated, levels of inducible NO are enhanced, leading to a release of adenosine in both the basal forebrain and the frontal cortex, in turn inhibiting basal forebrain and cortical wake-active neurons [279, 280.]
Cytokines. Cytokines are humoral factors that regulate both pathologic and normal functions including immune responses and sleep regulation [281–284]. The most well-studied cytokines are the pro-inflammatory molecules interleukin-1 beta (IL-1β) and tumor necrosis factor α (TNF-α), although many cytokines have been implicated in modulating sleep. Pro-inflammatory molecules typically enhance NREM sleep and SWA, while anti-inflammatory molecules attenuate these responses, demonstrated after enhanced waking activity or exposure to pathogens or other related molecules that enhance cytokines. Cytokines are present throughout the body including the brain and are produced by many brain cells types including neurons, microglia, perivascular macrophages, and astrocytes. Plasma and brain mRNA levels of IL-1β and TNF-α are elevated as the homeostatic drive for sleep is increased, such as occurs with sleep deprivation or spontaneous wake progression [285–287]. Administration of either of these cytokines enhances NREM sleep amounts and SWA in animal studies, and IL-1 systemic administration also induced sleep in humans [282–284, 288, 289]. Inhibition of cytokine production or knockout of either the IL-1 type 1 or TNF 55-kDa receptor in mice attenuated NREM sleep responses [290–295]. Extracellular ATP released by either immune cells, neurons or glia acts on the purine type 2 receptor, promoting glial release of both cytokines. In turn, these cytokines alter cell membrane properties allowing increased sensitivity to modulation by adenosine and neurotransmitters [282, 284].
Synaptic Homeostasis
Tononi and colleagues recently suggested that SWA provides a mechanism by which cortical synaptic homeostasis occurs, theorized in their Synaptic Homeostasis Hypothesis (SHY) [296–298]. As proposed, wakefulness involves strengthening of many synapses as the animal navigates and learns from its environment. These brain mechanisms demand increased metabolism, as energy stores are used. During SWA, synaptic downscaling occurs, as synaptic strength is downregulated and energy reserves restored, re-normalizing previously active synapses. Upregulation of the mRNA of a number of genes implicated in synaptic strengthening, including brain-derived neurotrophic factor (bdnf), neuronal activity-regulated pentraxin (narp), and activity-regulated cytoskeleton-associated protein (arc), was reported in animals killed immediately following wake, when compared to sleep mRNA profiles [299]. Ex vivo investigations analyzing the prefrontal cortex slice following sleep deprivation showed an increase in miniature excitatory postsynaptic currents (mEPSCs) in pyramidal neurons [300]. Investigations in drosophila demonstrated an increase in synaptic size and strength as flies were kept awake, and downscaling of synapses as sleep was allowed to proceed [301]. Cortical recordings in animals, as well as high-density EEG recordings in humans, demonstrate that the slope and amplitude of SWA diminishes as sleep progresses, which may also reflect synaptic downscaling [302–304].
Although SHY is an intriguing and valuable organizing hypothesis, there are many exceptions [45, 305]. For example, an increase in mEPSCs does not necessarily reflect synaptic strengthening due to neuronal firing. Many studies have shown an increase in synaptic strengthening mechanisms such as long-term potentiation during sleep, evident during NREM sleep spindles and REM sleep theta frequency oscillatory activity [306–312]. Recently, disrupted sleep was found to attenuate NREM branch-specific dendritic spine formation in the motor cortex of mice following motor learning, providing direct evidence that sleep promotes learning-dependent synapse formation [313]. Learning may demand both synaptic strengthening and weakening by such mechanisms as long-term depression, to allow extinction of non-critical forms of information [314, 315]. Also, most of all SHY-related investigations have evaluated synaptic strengthening in cortical regions, but not subcortical circuitry, such as that of the ARAS. Lastly, alternative mechanisms that may produce synaptic strengthening across the sleep/wake cycle, such as circadian fluctuation of temperature and glucocorticoids, need to be investigated [305].
Rem Sleep
EEG Profile of REM Sleep
REM sleep was first documented by Aserinsky and Kleitman in the 1950s [316, 317]. As shown in Fig. 5.1, the EEG profile of REM sleep is strikingly similar to that of wake, exhibiting cortical activation. Because of this similarity, this sleep state is also called “paradoxical sleep.” EMG recordings reveal a lack of postural control and little muscle tone compared to wake. EOG recordings capture the rapid lateralized eye movements that define this stage. Also evident during REM sleep are two EEG phenomena: pontine-geniculo-occipital waves, and theta rhythmicity, particularly in the hippocampus. During REM sleep and wake, bursts of GBO are often associated with theta activity in the hippocampus. Parvalbumin neurons in the hippocampus fire at gamma frequencies, and this activity may provide a mechanism for phase locking of GBO in the cortex to theta rhythms [12, 17].
Pontine-Geniculate-Occipital Cortex (PGO) Waves
PGO waves consist of field potential activity in the dorsal pons, lateral geniculate nucleus, and occipital cortex. These waves are recorded in animals immediately preceding transitions into REM sleep, as well as during REM sleep, and the source of activation appears to be in the reticular formation [45]. The dorsolateral pons, brachium conjunctivum, and subcoeruleus/sublaterodorsal tegmental nuclei all appear to be involved in PGO wave generation [318–320]. PET scans and field potential recordings in humans have noted increased activity in the right geniculate and occipital cortex during REM sleep [321, 322], and simultaneous fMRI and EEG recordings revealed REM-related activation in the pontine tegmentum, thalamus, and visual cortex [323].
Theta Rhythmicity During REM Sleep
The hippocampal theta rhythm consists of field oscillatory activity in the frequency range of 5–8 Hz and is seen throughout REM sleep, as well as during select tasks during wake, including attention, spatial navigation, and movement [47, 48, 324]. Theta generation originates in reticular formation sites, particularly the RPO, nucleus gigantocellularis, and pontis caudalis. These neurons fire tonically during theta and project to such regions as the supramammillary nucleus of the hypothalamus (SUM), where the incoming tonic barrage of activity is translated into phasic output that then impinges on such forebrain regions as MS/DBv in the basal forebrain. SUM projections also directly terminate on hippocampal regions [325]. MS/DBv then sends GABAergic and cholinergic projections to the hippocampus, where the field oscillatory theta rhythm is recorded.
REM Sleep-Related Neurotransmitters
Acetylcholine
Transection studies revealed that brain regions anterior to the midbrain and posterior to the medulla were not necessary for REM-related brainstem activity [326, 327], and later lesioning studies determined that in this loci, largely the dorsolateral pons, were nuclei necessary for REM sleep generation including the serotonergic dorsal and median raphe nuclei, noradrenergic LC, and cholinergic LDT/PPT [45, 328]. Unlike the majority of wake-related neurotransmitters, cholinergic neurons are uniquely active during both wake and REM sleep states. Levels of acetylcholine in the cortex, as well as choline acetyltransferase mRNA in the basal forebrain, are highest during REM sleep, compared to both wake and NREM sleep [52, 329].
Direct reticular formation injections of compounds that enhance cholinergic transmission such as carbachol, muscarinic agonists, or acetylcholinesterase inhibitors induce REM sleep [45]. Early studies using the cholinergic agonist carbachol in the cat demonstrated that reticular formation injections produced a REM-like sleep state [45, 330]. A similar induction of REM sleep can be induced in rats and mice, although the effect is not as robust, possibly due to species differences, difficulty of localization of the agent to the pontine reticular formation in smaller rodent brains, as well as differences in delivery methods [331–333]. Furthermore, non-specific lesioning of LDT/PPT attenuated REM sleep, and the amount of cholinergic cell loss in these tegmental regions strongly correlated with the amount of subsequent REM sleep loss [334]. c-Fos studies also indicate that LDT/PPT neurons are active during REM sleep [335, 336], although some investigators were unable to replicate these findings [114, 337]. Unit recordings reveal that a subpopulation of LDT/PPT neurons fire selectively during wake and/or REM sleep [69, 70, 196], and recent studies employing juxtacellular labeling and recordings demonstrated that many tegmental wake/REM-on cells were indeed cholinergic [71]. Also, cholinergic neurons of the basal forebrain fired in bursts in relation to hippocampal theta activity during both wake and REM sleep, and juxtacellularly identified cholinergic basal forebrain neurons were found to fire maximally during wake and REM sleep [53–56]. Cholinergic-specific lesioning in the basal forebrain produced a reduction in REM sleep individual bout duration, as the amplitude of theta activity was reduced [338]. It is likely that optogenetic and pharmacogenetic studies will greatly enhance our understanding of tegmental cholinergic neurons in REM sleep promotion.
GABA and Glutamate
Select GABAergic/galaninergic neurons in an extended cluster around the VLPO expressed c-Fos specifically during REM sleep, indicating that these neurons are active during REM sleep, and specific lesions to this region also produced REM sleep disturbance [114, 229]. Single unit recordings in the preoptic area revealed that select neurons fire preferentially during REM sleep, further confirming that a subpopulation of neurons in the preoptic region are REM sleep active [223, 224].
An area located near the cholinergic tegmental zone, the sublaterodorsal nucleus (SLD located ventral to the LC), also plays a role in promoting a major characteristic of REM sleep, muscle atonia. This area is called the perilocus coeruleus alpha in the cat, and either SLD or subcoeruleus nucleus in the rodent. Early investigations found that lesions in this region suppressed REM-related muscle atonia. In particular, larger lesions that included SLD, the amygdala and colliculus produced “oneric” dream-like behavior in the cat during REM sleep, including locomotion and head movement [339, 340]. GABAergic neurons in the pontine reticular formation most likely inhibit SLD activity during wake, and these neurons are themselves inhibited during REM sleep by means of tegmental cholinergic input [341–343]. Early work by Magoun and Rhines determined that a region in the ventral medulla in the vicinity of SLD played a role in muscle atonia during REM sleep, and later investigations described projections from SLD to a glycinergic cell population in the ventral medulla, which in turn projects to spinal cord motor neurons involved in postural muscle tone [344, 345]. More recently, Lu and colleagues proposed an alternative pathway, based on c-Fos and neuroanatomical tracer investigations, in which SLD sends a glutamatergic projection directly to GABAergic/glycinergic spinal cord interneurons, which in turn inhibit spinal cord motor neurons [114]. Recently, simultaneous blockade of both GABA-B and glycinergic/GABA-A receptors prevented REM sleep muscle atonia involving the trigeminal motor pool neurons [346, 347]. Therefore, brainstem GABAergic/glycinergic neurons promote atonia by inhibition of these motor neurons, as REM-active glutamatergic neurons excite these same GABAergic/glycinergic cell populations. It remains to be determined if similar mechanisms are at play involving other muscle atonia-related cell populations, such as hypoglossal motoneurons that innervate the genioglossal muscle or spinal cord motor neurons.
Melanin Concentrating Hormone
Interspersed among orexinergic neurons in the lateral hypothalamus are GABAergic neurons that secrete melanin concentrating hormone (MCH), and recent studies suggest that these neurons play a role in sleep regulation [348–351]. MCH acts to inhibit wake-active neuronal cell populations throughout the ARAS, including orexinergic neurons, allowing sleep to be expressed [350, 352, 353]. In turn, a number of wake-related brain nuclei may inhibit MCH activity. MCH neurons are largely inactive during wake, increase firing during NREM sleep, and fire maximally during REM sleep [354]. c-Fos assessed neuronal activation was also elevated in MCH neurons during sleep [355]. Intracerebroventricular injections of MCH significantly increased both NREM and REM sleep, and direct injections of MCH into wake-active nuclei such as RPO, dorsal raphe nucleus, or the basal forebrain promoted REM sleep [350]. MCH neurons act largely on the MCH1 receptor, localized on a number of ARAS-related nuclei. MCH1 receptor suppression by antagonists or genetic knockout produced decreased NREM and REM sleep amounts [349, 356–359]. Additionally, optogenetic stimulation of MCH neurons produced a significant decrease in wake, and excitation applied at the beginning of REM sleep extend REM sleep bout length [360]. Furthermore, optogenetic inhibition decreased REM sleep theta activity, although overall REM sleep amounts and bout lengths were largely unaffected [361].
The Reciprocal Interaction Model of REM Sleep Regulation
McCarley and Hobson introduced the reciprocal interaction model to explain the neural circuitry and neurotransmission involved in transitions to the REM sleep state, as well as the REM oscillator, which is about 90 min in humans and shorter in smaller animals [362]. A further goal was to have the model be mathematically correct in terms of describing these oscillations. Initially the model was purely based on Lotka-Volterra equations, originally proposed to describe predator/prey interactions. An update to the model included a more realistic limit cycle set of equations to account for circadian variation [363]. Other updates have included the growing neurophysiological knowledge of REM sleep control factors, including GABAergic influences on REM sleep regulation [45, 362, 363].
As proposed (Fig. 5.5), cholinergic LDT/PPT neuronal firing increases immediately preceding and during REM sleep. This cholinergic excitation promotes glutamatergic activity in reticular formation regions including the SLD, parabrachial nucleus, and RPO. Cholinergic activation, and excitatory effects on these glutamatergic populations, are responsible for many of the featured REM sleep characteristics reviewed here, including PGO waves, hippocampal theta activity, and muscle atonia. Cholinergic LDT/PPT neurons also inhibit select reticular formation GABAergic cell populations, whose role is to act as REM-off neurons, further promoting REM sleep. Both serotonergic and noradrenergic cell populations are REM-off and wake-on. Their activity during wake decreases significantly as REM sleep approaches due to autoinhibition, and they remain silent until the REM-wake transition [45]. The serotonergic dorsal raphe and noradrenergic LC both project to and inhibit LDT/PPT REM sleep-promoting activity. LDT/PPT cholinergic neurons excite GABAergic reticular formation populations which in turn inhibit both serotonergic and noradrenergic REM-off cell populations.


Fig. 5.5
Modified reciprocal interaction model of REM sleep control [362, 363] Blue nuclei and arrows are excitatory, and red inhibitory. Laterodorsal (LDT) and pedunculopontine (PPT) tegmental REM-on cholinergic activity excites reticular formation (RF) glutamatergic REM-on neurons. LDT/PPT REM-on neurons also excite GABAergic REM-on interneurons that act to inhibit dorsal raphe (DR) and locus coeruleus (LC) REM-off neuronal activity. LDT/PPT REM-on neurons also inhibit GABAergic REM-off interneuronal activity, in turn promoting REM-on RF neurons. As REM sleep progresses, REM-on cells begin to excite REM-off cells, leading to REM sleep cessation
It is important to note that LDT/PPT activity is comprised of both REM-on and wake–REM-on neurons (about 2/3) [69, 70, 364]. To test the hypothesis of differential inhibition of tegmental REM-on populations by aminergic neurons, Thakkar and collaborators developed a novel methodology in freely behaving animals allowing both extracellular single cell recording in PPT and local perfusion of neuropharmacological agents by way of an adjacent microdialysis probe [196]. Discharge activity of REM-on neurons was almost completely suppressed by local microdialysis perfusion of the selective 5-HT1A agonist 8-OH-DPAT, while this agonist had minimal or no effect on the wake/REM-on neurons. These findings suggest that REM sleep ends as the excitatory effects of REM-on neurons cause serotonergic and noradrenergic neurons to resume activity; this in turn, suppresses REM-on neuronal activity. In terms of further evaluation of the cholinergic influences on REM sleep using optogenetic or DREADD technology, it is important to note the presence of both REM-on and wake–REM-on neurons—important in EEG activation in both REM sleep and wake—in the cholinergic population. Activation of the entire population by optogenetic or DREADD experiments may thus fail to produce a clear-cut REM episode. We think early experiments that implicated the brainstem endogenous production of acetylcholine in REM sleep promotion by use of neostigmine are a helpful guide as to cholinergic influences on REM sleep, as microinjection of neostigmine into the pontine reticular formation of cats enhanced desynchronized sleep signs [365].
Summary
This chapter provides an overview of the neurobiology of vigilance state regulation. Over the last 50 years, both clinical and basic investigations have begun to elucidate the neural mechanisms regulating sleep, in part due to the development of exciting new experimental techniques. Increased understanding of these neural mechanisms will aid the development of pharmacological and behavioral treatment of sleep pathology.
Acknowledgments
This research was supported by the US Department of Veterans Affairs Medical Research Awards I01BX001356 (RWM) and IK2BX002823 (MRZ), NIH HL095491, NIH MH039683, and NIH MH016259.
References
1.
Committee on Sleep Medicine and Research, Institute of the National Academies (2006) Sleep disorders and sleep deprivation: an unmet public health problem. In: Colten HR, Altevogt BM (eds). National Academies Press, Washington, DC
2.
Mitler ME, Dement WC, Dinges DF (2000) Sleep medicine, public policy, and public health. In: Kryger MH, Roth T, Dement WC (eds) Principles and practice in sleep medicine. W.B. Saunders, Philadelphia, PA, pp 580–588
3.
Berger H (1930) Ueber das elektroenkelogramm des Menchen. J Psychol Neurol 40:160–179
4.
Davis H, Davis PA, Loomis AL, Harvey EN, Hobart G (1937) Changes in human brain potentials during the onset of sleep. Science 86(2237):448–450PubMed
5.
Loomis AL, Harvey EN, Hobart G (1935) Potential rhythms of the cerebral cortex during sleep. Science 81(2111):597–598PubMed
6.
Zhang F, Gradinaru V, Adamantidis AR, Durand R, Airan RD, de Lecea L et al (2010) Optogenetic interrogation of neural circuits: technology for probing mammalian brain structures. Nat Protoc 5(3):439–456PubMedPubMedCentral
7.
Boyden ES (2011) Optogenetics: using light to control the brain. Cerebrum 2011:16PubMedPubMedCentral
9.
Dong S, Allen JA, Farrell M, Roth BL (2010) A chemical-genetic approach for precise spatio-temporal control of cellular signaling. Mol BioSyst 6(8):1376–1380PubMed
11.
Lisman J (2005) The theta/gamma discrete phase code occurring during the hippocampal phase precession may be a more general brain coding scheme. Hippocampus 15(7):913–922
12.
Lisman JE, Jensen O (2013) The theta-gamma neural code. Neuron 77(6):1002–1016PubMedPubMedCentral
13.
Cardin JA, Carlen M, Meletis K, Knoblich U, Zhang F, Deisseroth K et al (2009) Driving fast-spiking cells induces gamma rhythm and controls sensory responses. Nature 459(7247):663–667PubMedPubMedCentral
14.
Deco G, Thiele A (2009) Attention: oscillations and neuropharmacology. Eur J Neurosci 30(3):347–354PubMedPubMedCentral
15.
Sohal VS, Zhang F, Yizhar O, Deisseroth K (2009) Parvalbumin neurons and gamma rhythms enhance cortical circuit performance. Nature 459(7247):698–702PubMedPubMedCentral
16.
Li N, Wang Y (2008) M. W, Liu H. Effects of sleep deprivation on gamma oscillation of waking human EEG. Prog Nat Sci 18:1533–1537
17.
Buzsaki G, Wang XJ (2012) Mechanisms of gamma oscillations. Annu Rev Neurosci 35:203–225PubMedPubMedCentral
18.
Bragin A, Engel J Jr, Wilson CL, Fried I, Buzsaki G (1999) High-frequency oscillations in human brain. Hippocampus 9(2):137–142PubMed
19.
Puig MV, Ushimaru M, Kawaguchi Y (2008) Two distinct activity patterns of fast-spiking interneurons during neocortical UP states. Proc Natl Acad Sci U S A 105(24):8428–8433PubMedPubMedCentral
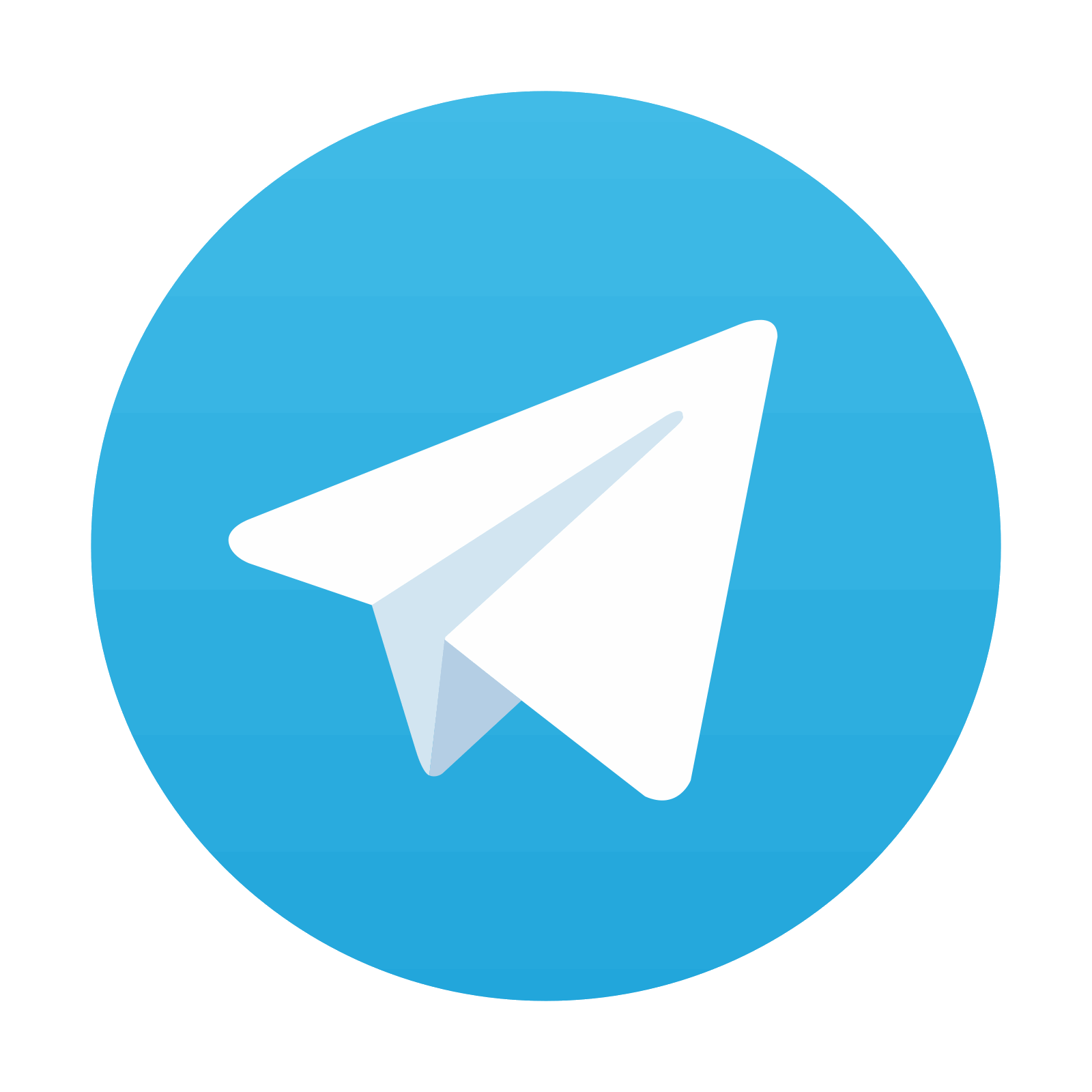
Stay updated, free articles. Join our Telegram channel
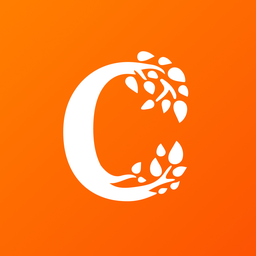
Full access? Get Clinical Tree
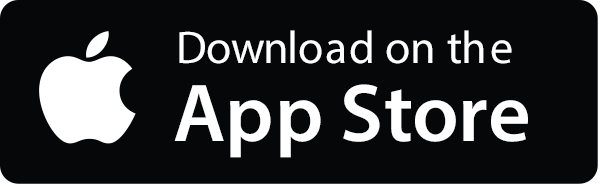
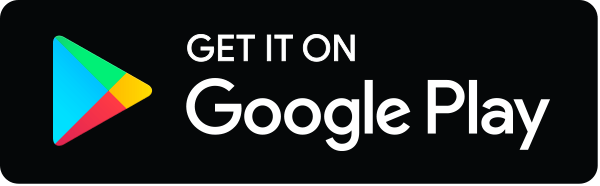