Neurochemistry, Pharmacodynamics, and Biological Psychiatry
George M. Anderson
Andrés Martin
Introduction
Continuing advances in basic neurobiology and psychopharmacology have led to a greatly expanded knowledge of brain functioning and hold the promise of better treatment and fuller understanding of childhood psychiatric disorders (1,2). The recent elucidation of the genetic bases of a number of single-gene childhood psychiatric disorders provides additional hope, and to some extent directions, for tackling the more complex molecular and biological influences in autism, attention deficit/hyperactivity disorder (ADHD), Tourette syndrome, anxiety, posttraumatic stress disorder (PTSD), depression, and suicide. Identification of causative factors in Parkinson’s disease, Huntington’s disease and Alzheimer’s disease further encourages the notion that the pharmacology of childhood psychiatric disorders can be made more rational and effective, and the biological bases of the relevant behaviors ascertained.
The three entwined areas of neurobiology, pharmacodynamics, and biological psychiatry can be introduced by first considering basic concepts in the separate realms of inquiry. Relevant neurobiology includes findings in the areas of neuronal circuitry, neural transmission and intracellular signaling. Phamacodynamics concerns the short- and long-term effects of drugs on neuronal function and structure. Insights and serendipitous findings from psychopharmacology reciprocally inform basic neuroscience and also have often been central to the hypotheses pursued in biological psychiatry. Recognition of the importance of genetics and complexity of psychiatric disorders is changing in fundamental ways how biological psychiatry is approached. Whether the field is termed molecular psychiatry, biological psychiatry, or clinical neuroscience, an increased focus on genetic influences and on behavioral components or endophenotypes has been prompted by a better appreciation of the scope and complexity of the endeavor.
General Considerations of CNS Functioning
Overview
Neuronal circuitry, synaptic neurotransmission, and intracellular information processing constitute three major levels of central nervous system (CNS) functioning critical to understanding mechanisms of psychotropic drug action and the biological basis of cognitive and behavioral processes.
Neuronal circuitry to a large extent defines and reflects the functional activity and organization of the CNS. Neuronal communication via neurotransmitter release is a fundamental mechanism of brain function. The release of neurotransmitters and neuromodulators, their mechanisms of action, and their effect on target neurons are complex and still not fully understood; however, despite the diversity of neurotransmitters and receptors in the human brain, all forms of neural communication have the common goal of modulating neuronal activity. This is achieved by changing either the electrical or biochemical properties of the cell. The balance of intracellular and extracellular ions characterizes the electrical properties of the neuron. At rest, there are more negatively charged ions inside than outside the cell, thereby creating a negative resting membrane potential. Decreasing the resting potential leads to excitation, increasing it, to inhibition. The more enduring properties of the neuron are determined by longer term processes regulating the expression of specific genes, the production of proteins, and the creation of a distinct metabolism.
Neuronal Circuitry
The adult human cerebral cortex has about 100 billion neurons; each neuron establishes about a thousand to ten thousand connections to other neurons. Neurons are arranged in distributed networks that play critical roles in the expression of human behavior (3). This involves the collection of sensory information through perceptual modules, the creation of a representation, and the production of a response. Here we focus on four major anatomic systems that are crucial for these three steps of information processing: the cortex, thalamus, basal ganglia, and medial temporal lobe (Figure 2.4.2.1).
The thalamus is the gateway to cortical processing of all incoming sensory information, here represented by the three major systems: somatosensory, auditory, and visual (Figure 2.4.2.1). Primary sensory cortices receive information from the appropriate input modules (sensory organ + thalamus).
The association cortex integrates information from primary cortices, subcortical structures, and brain areas affiliated with memory to create an internal representation of the sensory information.
The medial temporal lobe (including the hippocampus and amygdala) serves two major functions in the brain: to integrate multimodal sensory information for storage into and retrieval from memory, and to attach limbic valence to sensory information (e.g., pleasant or unpleasant, fight or flight).
The basal ganglia are primarily involved in the integration of input from cortical areas. The basal ganglia modulate cortical activity via a cortico-striato-pallido-thalamo-cortical (CSPTC) loop. The most prominent projections to the striatum arise from the motor cortex.
All major projections (solid lines) in this basic circuitry (Figure 2.4.2.1) are glutamatergic (using the amino acid neurotransmitter glutamate), except for the projections from the basal ganglia toward the thalamus, which are GABAergic (employing γ-aminobutyric acid). The glutamatergic neurons within each of the major components or regions of the circuit are under inhibitory control by GABAergic interneurons. In addition, four groups of densely packed neurons provide diffuse projections to all areas of the brain to modulate their functions: cholinergic neurons in the basal forebrain and brain stem, dopaminergic neurons in the substantia nigra and ventral tegmental area, noradrenergic neurons in the locus ceruleus, and serotonergic neurons in the raphe nuclei. The broken arrows in Figure 2.4.2.1 indicate the four neurotransmitter-specific projection systems. The relay of information from one neuron to another in these various circuits is usually effected by synaptic neurotransmission.
General Aspects of Synaptic Neurotransmission
Dendrites create a network of fibers providing the cell body of the neuron with input from other cells (Figure 2.4.2.2). The cell integrates these different inputs through modulation of the membrane potential, changes in second messenger systems and at the level of the nucleus (regulation of gene expression). The cell body is also the site of synthesis of nearly all cell-specific proteins, including transporters, receptors, and the enzymes needed for neurotransmitter production.
The axon is the output station of the neuron. The axon can be short (local circuit neuron) or long (projection neuron). If a deviation from the resting membrane potential is above a certain threshold, an action potential is created and travels downstream rapidly. The nerve terminal is the widened terminal part of the axon. It provides a small area of close contact with dendrites of communicating neurons: the synapse. Variations of this typical scheme include synapses between two terminals, two dendrites, and neurotransmitter release in medial parts of the axon.
As seen in Figure 2.4.2.2, the presynaptic neuron releases the vesicular stored neurotransmitter into the synapse and can express two types of proteins that affect synaptic communication: Presynaptic membrane-bound receptors can bind the intrinsic neurotransmitter (at autoreceptors) or transmitters of neighboring neurons (at heteroreceptors) and
affect the cell via intracellular messengers. One response, for example, is the modulation of neurotransmitter release (4). Presynaptic membrane-bound reuptake transporters pump the released neurotransmitter back into the cell (5). The released transmitter can undergo intracellular, extracellular metabolism (4B), or be repackaged in vesicules for rerelease.
affect the cell via intracellular messengers. One response, for example, is the modulation of neurotransmitter release (4). Presynaptic membrane-bound reuptake transporters pump the released neurotransmitter back into the cell (5). The released transmitter can undergo intracellular, extracellular metabolism (4B), or be repackaged in vesicules for rerelease.
The neuron receiving the input (postsynaptic cell) can be modulated via two different types of receptors (Figure 2.4.2.2). In the case of fast-acting, class I (ionotropic) receptors, the neurotransmitter binds to the receptor protein and within milliseconds this leads to a change in the permeability of the associated ion channel, allowing the influx of ions such as Ca2+, Na+, K+, or Cl–. In contrast, with slow-acting, class II (G-protein-coupled) receptors, the binding of the neurotransmitter to the receptor protein leads to a change in the protein conformation. This change is relayed to an associated G-protein, so called because it binds guanidine triphosphate (GTP) in order to be activated. G-proteins regulate two major classes of effector molecules: ion channels and second messenger generating enzymes. This general pattern and form of synaptic neurotransmission is repeated across the six major neurotransmitters. However, the differences seen across the glutamatergic, GABAergic, dopaminergic, nor- adrenergic, serotonergic and cholinergic systems are intriguing, form the bases of specific psychopharmacological effects, and underlie still prevailing neurobiological theories of biological psychiatry.
Intracellular Information Processing
Our understanding of how neurons relay information between each other has moved beyond the role of synaptic transmission and into that of processes that take place within cells. Each neuron is the target of many projections from local and distant neurons. These influences are integrated at the level of the cell membrane and cell nucleus. The neurotransmitter-mediated activation of ion channels in the cell membrane can lead to an increase or decrease of the resting membrane potential. This may lead to the creation of an action potential. At the level of the cell nucleus, the various receptors and ion channels expressed on the cell membrane may influence gene and protein expression.
Gene expression is regulated by transcription factors that bind to specific sequences of the DNA in the nucleus (Figure 2.4.2.2 B); therefore, membrane-bound receptors or ion channels in distal parts of the neuron must be able to activate intraneuronal signal transduction pathways that can span long distances and translocate to the nucleus. Because proteins assemble the neuron and determine neuronal properties, gene expression regulates neuronal function and may cause malfunction. Many psychopharmacologic agents with delayed therapeutic effects are thought to produce their therapeutic benefits through modulation of gene expression (6,7).
Release of neurotransmitters from the presynaptic neuron into the synapse activates receptors on the postsynaptic neuron (see Figure 2.4.2.2 B). Ions such as calcium enter the cell and act as second messengers on activation of inotropic receptors. Activation of G-protein–coupled receptors facilitates the opening of neighboring ion channels or the synthesis of second messengers such as cyclic AMP. Second messengers (calcium, cyclic AMP) regulate the activity of protein kinases (proteins that transfer phosphate groups to a substrate protein) and phosphatases (proteins that remove phosphate groups from a substrate protein). In all cases investigated to date, the activation of neurotransmitter receptors changes the state of phosphorylation of neuronal proteins.
The transcription factors are one group of proteins regulated by phosphorylation. Transcription factors operate by recruiting the transcription initiation complex and RNA polymerase to particular genes. The RNA polymerase then transcribes the DNA template into an RNA molecule, which is translated into protein outside the nucleus.
Among the best-studied transcription factors in the brain is the Ca2+– and cyclic AMP-responsive element binding protein (CREB). The study of CREB has provided us with an insight into the complex consequences of transcription factor activation and gene expression on higher brain function. A variety of signal transduction pathways are integrated into CREB-mediated gene expression, such as those activated by Gs-proteins, inotropic receptors, or growth factors. Cyclic AMP-responsive element binding protein is activated by phosphorylation and regulates the expression of several target genes (e.g., genes for peptide neurotransmitters, enzymes involved in neurotransmitter synthesis, and growth factors). The discovery that CREB plays a vital role in processes such as learning and memory provided a link between gene regulation and cognitive function.
Small but persistent abnormalities in neurotransmission can have far-reaching consequences because neurotransmitters and receptors influence gene and protein expression in the brain. An understanding of signal transduction pathways and transcription factors such as CREB may be instrumental in providing new therapeutic avenues in psychopharmacology.
Pharmacodynamics Overview
Pharmacodynamic principles are concerned with the biochemical and physiologic effects of drugs at their active sites, that is, with their specific mechanisms of action. Stated succinctly, “pharmacokinetics describes what the body does to a drug; pharmacodynamics what a drug does to the body” (see Chapter 6.1.1 Oesterheld, Shader & Martin). The effects of a medication may change during development, as brain regions or neurotransmitter systems develop and mature. Most psychopharmacological agents exert their effects by interacting with specific protein targets— receptors, ion channels, transporters, or enzymes. Each of the major neurotransmitter systems have, therefore, a number of routes by which they can be manipulated (as are delineated in subsequent sections). The nature of the drug effect depends upon the site targeted while the selectivity of action is often a function of the relative affinity of the agent for the target site versus its other, often multiple, sites of interaction. Adverse drug effects are often the result of relatively nonselective agents and can severely limit their clinical utility.
Biological Psychiatry Overview
Research in biological psychiatry has made it increasingly clear that the genetics and biology of the common disorders are complex, in that multiple genes appear to contribute to the syndromes (Chapters 2.3.2 Fernandez & State and 2.3.1 Leckman, Lombroso & Vaccarino). This is not surprising given the complex and diffuse nature of the neural systems that subserve the relevant brain processes (8). The genetic complexity is further multiplied by the wealth of variation arising from gene–environment interactions: Illuminating the complexity will require the application of new and appropriate genetic methods (9,10,11).
An emerging overarching theme is that all biological, neuropharmacologic, and behavioral investigation needs to be performed in a genetic context. There is also a greater focus on components or domains of behavior and a greater interest in quantifying the traits or variables of interest. The rationale for examining what have been referred to as elementary units of psychological dysfunction (12), core or candidate symptoms, quantitative phenotypes (13), endophenotypes (14), or core psychopathological processes (15,16) is becoming more and more compelling.
One formulation of the interrelated aspects of biological psychiatry is presented in Figure 2.4.2.3. The mutual interacting influences of genetic and environmental factors
are shown determining the neurobiological systems that, in combination, form the substrate of relevant behaviors. The field of biological psychiatry has traditionally emphasized neurochemical and neuroendocrinologic approaches. Other fields that are now proving critical to advancing an understanding of brain neural transmission in psychiatry include neurophysiology, neuropsychology, neuroimaging (17) and pharmacogenetics (18). Elucidation of the bases of the childhood disorders can be expected to proceed through an iterative process involving mutually beneficial relationships among all of the perspectives (19,20). It is clear that one should be ever mindful of the developmental context when studying childhood psychiatric disorders (21,22).
are shown determining the neurobiological systems that, in combination, form the substrate of relevant behaviors. The field of biological psychiatry has traditionally emphasized neurochemical and neuroendocrinologic approaches. Other fields that are now proving critical to advancing an understanding of brain neural transmission in psychiatry include neurophysiology, neuropsychology, neuroimaging (17) and pharmacogenetics (18). Elucidation of the bases of the childhood disorders can be expected to proceed through an iterative process involving mutually beneficial relationships among all of the perspectives (19,20). It is clear that one should be ever mindful of the developmental context when studying childhood psychiatric disorders (21,22).
Neurotransmitter Systems
Overview
The major neurotransmitter systems can be divided into two groups based on their anatomic distribution. The first group comprises the serotonergic, dopaminergic, noradrenergic and cholinergic neurons. These four systems originate from small groups of neurons, densely packed in circumscribed areas of the forebrain or brain stem, and project to their target areas typically by long-ranging projection fibers. The second group includes the glutamatergic and GABAergic systems. Their neurons are by far the most prevalent and widely distributed types in the human brain. As mentioned, a number of similarities and parallels are apparent across the neurotransmitter system. Each system will be considered from the standpoint of its anatomy and neural transmission mechanisms, its pharmacodynamics or psychopharmacology, and the relevant biological psychiatry investigation.
Serotonergic System
Serotoninergic Neural Transmission/Neuroanatomy
Most serotonergic cells overlap with the distribution of the raphe nuclei in the brainstem. A rostral group (B6–8 neurons) projects to the thalamus, hypothalamus, amygdala, striatum, and cortex (23). The remaining two groups (B1–5 neurons) project to other brainstem neurons, the cerebellum, and the spinal cord. A schematic diagram of a serotonergic neuron and the metabolism of serotonin (5-HT) are depicted in Figure 2.4.2.4. Serotonin is produced after hydroxylation and decarboxylation of the essential amino acid tryptophan. Serotonin produced can be taken up by the vesicular monoamine transporter (VMAT2) and stored in vesicles for subsequent release or metabolized by the mitochondrial enzyme monoamine oxidase (MAO). Once released, 5-HT can interact with presynaptic or postsynaptic receptors, diffuse to extrasynaptic sites, or be taken up by neuronal or glial membrane 5-HT transporters (5-HTTs).
Serotonin acts at two different classes of receptors: at an inotropic receptor (5-HT3 receptor) or at slower acting
receptors, coupled either to phospholipase C (5-HT2 receptors) or to G-proteins (5-H T1,4–7 receptors) (24). The 5-HT1 receptors (5-HT1A,B,C,D,E,F) (Figure 2.4.2.4) are coupled to Gi and lead to a decrease of cyclic AMP. The 5-HT1A receptor is also directly coupled to a K+ channel leading to increased opening of the channel. The 5-HT1 receptors are the predominant serotonergic autoreceptors. 5-HT2 receptors (5-HT2A–C) are coupled to phospholipase C and lead to a variety of intracellular effects (mainly depolarization). Three receptors (5-HT4, 6 & 7) are coupled to Gs and activate adenylate cyclase. The 5-HT3 receptor is the only monoamine receptor coupled to an ion channel, and is found in the cortex, hippocampus, and in the area postrema, where it mediates nausea and emesis. It is typically localized presynaptically and regulates neurotransmitter release.
receptors, coupled either to phospholipase C (5-HT2 receptors) or to G-proteins (5-H T1,4–7 receptors) (24). The 5-HT1 receptors (5-HT1A,B,C,D,E,F) (Figure 2.4.2.4) are coupled to Gi and lead to a decrease of cyclic AMP. The 5-HT1A receptor is also directly coupled to a K+ channel leading to increased opening of the channel. The 5-HT1 receptors are the predominant serotonergic autoreceptors. 5-HT2 receptors (5-HT2A–C) are coupled to phospholipase C and lead to a variety of intracellular effects (mainly depolarization). Three receptors (5-HT4, 6 & 7) are coupled to Gs and activate adenylate cyclase. The 5-HT3 receptor is the only monoamine receptor coupled to an ion channel, and is found in the cortex, hippocampus, and in the area postrema, where it mediates nausea and emesis. It is typically localized presynaptically and regulates neurotransmitter release.
Serotonergic Pharmacodynamics
Serotonin is linked to many brain functions because of the widespread serotonergic projections and heterogeneity of the serotonergic receptors (23,25). For example, modulation of serotonergic receptors and the reuptake site is beneficial (among others) in the treatment of anxiety, depression, obsessive-compulsive disorder, and schizophrenia (26). Interest in central 5-HT functioning derives from 5-HT’s important role in processes as diverse and important as sleep, mood, appetite, perception, and hormone secretion (25), as well as its critical role in neurodevelopment (27).
Blockade of the serotonin transporter by selective serotonin reuptake inhibitors (SSRIs), such as fluoxetine (Prozac), results in higher levels of synaptic and extracellular fluid 5-HT and leads to greater pre- and postsynaptic 5-HT receptor stimulation. The serotonin transporter is the primary target site for several antidepressants, including the selective serotonin reuptake inhibitors (SSRIs), venlafaxine, and tricyclic antidepressants such as clomipramine and, to a lesser extent, imipramine and amitriptyline (Figure 2.4.2.4). Recent work in nonhuman primates has indicated that the increase in functionally active 5-HT occur within hours and is relatively constant over the course of treatment.
The atypical neuroleptics, such as risperidone, ziprasidone, and olanzapine all act as antagonists at 5-HT2 receptors, as well as having blocking properties at dopamine receptors. The beneficial antipsychotic effects of the drugs appear to be at least partly mediated through their effects at cortical 5-HT2A receptors. In particular, it appears that 5-HT2A receptors on apical dendrites of cortical pyramidal cells may be especially important in gating sensory input. The critical role of the 5-HT2A receptor in perception is underscored by a consideration of the effects of the 5-HT2A agonist lysergic acid diethylamide (LSD). Adverse effects of the atypical neuroleptics on appetite and the associated weight gain appear to be at least partly due to effects at the 5-HT2C receptor, while the substantial and enduring hyperprolactinemia frequently observed is a consequence of the dopamine D2 receptor blockade in the pituitary.
Other serotonergic agents include the atypical anxiolytic buspirone, which acts as an agonist at the 5-HT1A receptor. Newer antimigraine drugs such as sumatriptan have agonist effects at arterial 5-HT1B/D receptors, while the antiemetic odansetron acts to antagonize 5-HT3 receptor sites in the intestine and perhaps in the brain.
Biological Psychiatry of Serotonin
Autism
Initial interest in a role for 5-HT in autism stemmed in part from the powerful effects of serotonergic agents, such as LSD, on perception. Research in the area was further stimulated by early reports of elevated 5-HT in blood of autistic children (28,29). Beneficial effects of treatment with serotonin reuptake inhibitors (30) and exacerbation observed after tryptophan depletion (31) have also heightened interest in the role of 5-HT in autism. Although most of the 5-HT-related research has focused on the hyperserotonemia of autism, a number of studies of CSF 5-HIAA and several neuroendocrinologic studies of central 5-HT functioning have been reported (32). CSF studies are in general agreement that few or no differences exist between autistic and control groups’ mean levels of 5-HIAA (33). A series of studies has provided converging evidence that 5-HT2A receptor function and expression may be altered in autism. In 1989, McBride and colleagues reported that central and peripheral 5-HT2A receptor functioning appeared reduced in autism (34). Thus, a diminished 5-HT2A -mediated neuroendocrine response was paralleled by a reduced 5-HT2A -mediated platelet aggregation response and lower platelet 5-HT2A receptor binding in autism. Alterations in platelet 5-HT2A binding indices were also reported by Cook et al. (35), with 5-HT2A receptor measures inversely related to platelet 5-HT levels. These reports are paralleled by two neuroimaging studies reporting reduced 5-HT2A receptor density in cortical regions (36).
TS/OCD
A role for 5-HT has been hypothesized because of the close connection between TS and obsessive-compulsive disorder (37). Effective treatment of obsessive-compulsive symptoms in patients with TS with the serotonergic uptake inhibitors fluvoxamine and fluoxetine has further stimulated research on the connection. The largest study of the 5-HT metabolite, 5-hydroxyindoleacetice acid (5-HIAA), found similar levels in patients with TS, OCD, or TS plus OCD, and the normal control group (38). No studies of 5-HT receptor functioning have been carried out in patients with TS, and early reports of benefit from the immediate 5-HT precursor, 5-hydroxytryptophan, have not been replicated. Research examining postmortem brain tissue has found decreases in 5-HT, 5-HIAA, and tryptophan across nearly all cortical and subcortical areas in TS (39,40). Further post-mortem research is necessary in order to replicate the findings; however, the results tend to increase the possibility that 5-HT may be a factor in the symptomatology of TS.
ADHD
Interest in a role for 5-HT in ADHD was stimulated by early reports of decreased platelet 5-HT in affected children. Subsequent studies have not replicated this finding and have found normal levels of platelet and urine 5-HIAA, as well as normal numbers and affinities of platelet imipramine-binding sites in subjects with ADHD. In addition, studies of CSF 5-HIAA have not found differences between ADHD and control subjects. On the whole, the neurochemical research and the minimal treatment response to serotonergic agents have made it seem less likely that a 5-HT alteration is etiologic. Even so, it appears that the role of 5-HT in disruptive behaviors, particularly with respect to impulse control, deserves further consideration.
Dopaminergic System
Dopaminergic Neural Transmission/Neuroanatomy
Dopaminergic neurons can be divided into three major groups based on the length of their efferent fibers: a) ultrashort systems in the retina and olfactory bulb; b) intermediate-length systems originating in the hypothalamus and projecting to the pituitary gland; and c) wide-ranging systems originating from
two areas, the substantia nigra (SN) and the ventral tegmental area (VTA). The SN neurons (also called A9 neurons) project to caudate and putamen, whereas the VTA neurons (also called A10 neurons) project to limbic areas such as nucleus accumbens and amygdala (i.e., mesolimbic projections) and several cortical areas such as frontal, cingulate, and entorhinal cortex (i.e., mesocortical projections).
two areas, the substantia nigra (SN) and the ventral tegmental area (VTA). The SN neurons (also called A9 neurons) project to caudate and putamen, whereas the VTA neurons (also called A10 neurons) project to limbic areas such as nucleus accumbens and amygdala (i.e., mesolimbic projections) and several cortical areas such as frontal, cingulate, and entorhinal cortex (i.e., mesocortical projections).
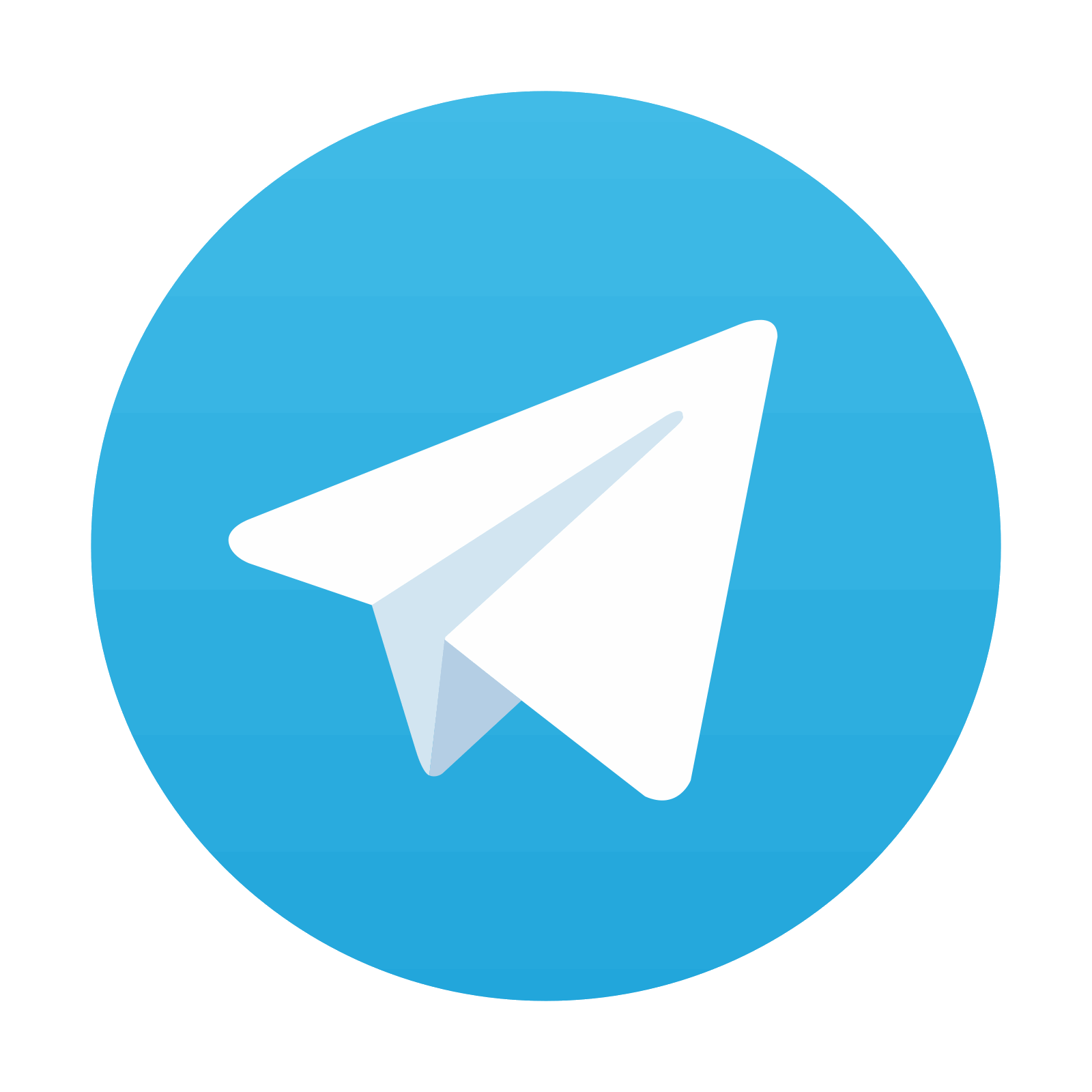
Stay updated, free articles. Join our Telegram channel
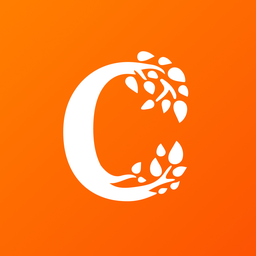
Full access? Get Clinical Tree
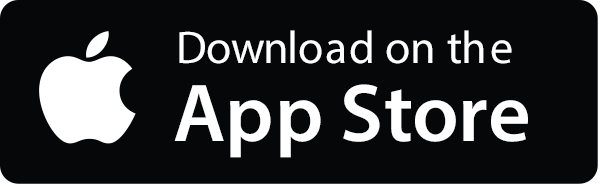
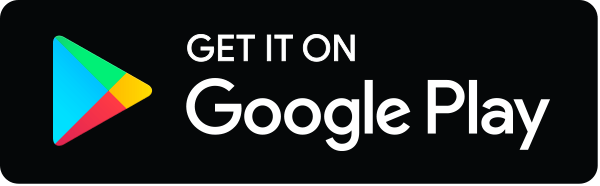