Fig. 3.1
Shunt series in a 14-year-old patient with a Strata VP shunt: (a, b) skull, (c) chest, and (d) abdomen radiographs showing the integrity of the shunt
To facilitate radiographic evaluation, the catheter and distal tubing are manufactured with either barium impregnation, which allows visualization of the entire tube, or they may be endowed with intermittent radiopaque markers along their course or tips. The valve itself is almost always entirely radiopaque [7]. Disconnections of VP shunt mechanism are a recognized complication and occur most commonly at the level of the valve, where the proximal and distal tubing meet [8]. Similarly, actual breaks in the catheter tubing are more likely to occur in the neck, likely owing to its increased mobility [7].
Other plain radiographic findings include the ectopic position of the shunt catheters. The tubing may also be coiled, causing shunt malfunction, which is a readily detectable plain radiographic finding. Occasionally, development of an abdominal pseudocyst or abdominal catheter adhesions might be suspected on plain radiography by the observation of a fixed, static position of the distal catheter tip on subsequent studies [3, 7].
Briefly, catheter obstruction may not produce any findings on plain radiographs; consequently, the value of shunt series is further limited to demonstrating disruptions of the system, including disconnections and kinks [7]. Some authors have demonstrated that the shunt series have a sensitivity lower than 19.4 %, while others suggest that the true sensitivity is not higher than 31 %. Plain radiographic examination has a low sensitivity and significant false-negative rates for detecting shunt abnormalities. In addition, the existence of many possible causes of shunt failure, together with the use of ionizing radiation, limits the role of the shunt series to identifying potential mechanical causes [2, 7].
The estimated radiation dose from a plain skull, chest, and abdomen radiograph is 525 millirem (5.28 mSv). This radiation dose is approximately equivalent to 2 years of background radiation. If a mechanical cause of failure is suspected, it might be possible to obtain plain radiographs using lower mA settings, without losing diagnostic information for evaluating the catheter components [7]. The radiographic shunt series provides critical information for operative planning, and some authors propose that this study may be better employed after the decision of a surgical revision of the shunt has been taken, based on clinical grounds, CT, MR, and/or nuclear imaging [6].
3.2.2 Ultrasounds
Transfontanelar US are used to explore the brain and the ventricles, but its use is limited to the first 12–18 months of life when the anterior fontanel is still open (Fig. 3.2). Ultrasonography can be used as a bedside screening test. The size and shape of the lateral ventricles are easily visualized, but the size of the third and fourth ventricles sometimes is difficult to assess by this technique. Therefore, the precise diagnosis and cause of hydrocephalus are rarely made by US alone. Furthermore, US are usually unable to show details of the posterior fossa, the aqueduct, the third ventricle floor, and the foramen magnum. Besides, the quality of the US image is user dependent, and its reproducibility is not high. Accordingly, the best indication for US is the follow-up of ventricular dilatation, before or after surgical treatment, limiting its use to the first 2 years of life. In case that surgical treatment of ventriculomegaly is indicated, other means of brain imaging are ordinarily obtained [5, 8, 9].


Fig. 3.2
Transfontanelar US in post-hemorrhagic hydrocephalus: (a, b) a 29-gestational-week newborn with grade III hemorrhage. (c) Coronal and (b) sagittal cuts showing hydrocephalus with a left intraventricular clot. (c, d) A 27-gestational-week neonate given a Polaris valve for progressive hydrocephalus illustrating suture straddling and clinical suspicion of overdrainage. Coronal views demonstrating (c) ventricular collapse and (d) the intraventricular valve
Abdominal US is frequently used to assess the peritoneal end in VP shunts and to demonstrate its complications as are an abnormal position of the distal catheter, CSF pseudocysts, etc. Thoracic US can also be indicated in the evaluation of pleural fluid in ventriculopleural shunts (though they are rarely used because of frequent pleural effusions) [2, 10].
3.2.3 Computerized Tomography
CT is the best method for imaging the brain as it is easily available, reliable, and compatible with standard life support devices [8]. CT is often the preferred technique (especially in emergencies) because of its wide availability, ease of use, and brief imaging time [11]. CT of the head is also commonly utilized for follow-up examinations as subsequent CT studies can be easily compared. Children often do not necessitate to be sedated. In the case of shunt obstruction, the nature and site of the occlusion can be easily viewed with CT that shows ventricular dilatation proximal to the block, accompanied by normal-sized or compressed ventricles distal to it, although not with sufficient detail. CT is also very useful in the follow-up of patients after initial shunt placement (Fig. 3.3). The ventricular size usually decreases gradually from the time of shunt insertion up to 12 months, and then it often remains stable. Most authors consider that the 1-year follow-up CT head scan is the most useful baseline study for long-term follow-up [5].


Fig. 3.3
Post-hemorrhagic hydrocephalus patient with Polaris valve. Simple cranial CT in the axial plane shows hydrocephalus and the intraventricular catheter well positioned in left frontal horn (a,b). In 3D Volumen Rendering reconstruction (VR) (c,d) the intraventricular catheter, reservoir, valve and distal catheter are seen
However, taking into account that most CSF shunt failures appear soon after initial shunting and that they are more common during the first year, some authors recommend to obtain a 3-month scan. In addition, young children need a lifelong follow-up, and also they often require frequent CT studies for assessment during episodes of suspected shunt malfunction [9, 12]. The most important concern about CT follow-up studies in shunted children is the repeated use of ionizing radiation and its consequent potential cumulative risk for developing cancer later in life [5]. Steps to minimize the risk for possible long-term complications of ionizing radiation are, therefore, particularly relevant in children undergoing multiple follow-up CT scans. Low-dose CT protocols are being designed to balance image quality and radiation dose [11].
3.2.4 Magnetic Resonance Imaging
MRI achieves similar structural findings than CT and is being increasingly used to reduce radiation exposure [4]. MRI is the examination of choice for showing ventricular dilatation, being capable of differentiating ventriculomegaly from hydrocephalus, and can also reveal its underlying cause [8].
Significant MRI-induced heating has not been shown to be a problem in recent models of shunt valves. An external magnet can adjust pressure settings of modern programmable valves. Changes in valve pressure settings often occur after exposure of these valves to MRI magnetic fields [13]. Before performing a MRI examination to a patient harboring a programmable valve, the radiologist must confirm if the shunt is or is not resistant to reprogramming by the magnetic field strength of the scanner. After scanning, the neurosurgeon will usually check whether the valve pressure setting has changed using a compass provided by the valve’s manufacturer [4, 14]. Newer valves, such as the Polaris and Pro-GAV, are resistant to accidental reprogramming even at 3-T magnetic fields, and do not require adjustments of the pressure settings of the device after a MRI study. In case of having to perform a MRI to a patient with an unfamiliar valve, the radiologist must check the patient’s written clinical report about the type of valve or contact the manufacturer (or representative) for specific MRI safety guidelines.
At present, the normal anatomy of CSF pathways, and the function of CSF drainage systems, demands a detailed study. For this purpose, new MRI sequences, either alone or in combination, are obtained in addition to conventional T1- and T2-weighted images. Although various MR cisternography and motion-sensitive MRI techniques are utilized, 3D CISS or its equivalent, TSE or FSE, and cine PC have gained wide acceptance in evaluating CSF flow and in depicting the anatomy of the cisterns [15, 16].
3.2.4.1 Conventional Sequences
T1- and T2-weighted are the sequences routinely used. Both are quite informative for demonstrating pathologic signal intensities within the brain parenchyma and for the assessment of ventricular shape and size. In addition, these sequences show most of the space-occupying lesions in both intra- and extra-axial locations (Fig. 3.4) [8]. Conventional MRI also provides useful information about the etiology of the hydrocephalus. Nevertheless, these criteria depend on a subjective evaluation by the neuroradiologist that may render the images difficult to assess in some instances, and thus they may be hardly comparable in postsurgical studies [17, 18]. The demonstration of the presence or absence of hemorrhage and of related membranes in the cisterns by using hemosiderin-sensitive sequences under the age of 2 years is also essential. Gradient echo (GRE) T2* or susceptibility weighted imaging (SWI) easily detects previous hemorrhage in the ventricles and cisterns.




Fig. 3.4
Control cranial MR of a 7-month-old patient with a Polaris valve showing an artifact caused by the intraventricular catheter in axial FSE T2 (a), FSE T1 (c), b1500 DWI (d), and gradient-echo (e) sequences. Axial T2 plane shows the intraventricular catheter (b)
3.2.4.2 Three-Dimensional Constructive Interference in the Steady State
Three-dimensional constructive interference in the steady state (3D-CISS) is a gradient-echo imaging technique with high resolution that remains sensitive to flow and provides fine anatomic details of the CSF pathways. 3D-CISS enables locating the obstruction and determining the upstream impact. It provides anatomical information about third ventricle morphology and its relationships, details that are extremely useful before performing an ETV. These sequences also allow for a good visualization of the cerebral aqueduct and for the diagnosis of the cause of obstruction, sometimes better than with classical sequences [8, 15, 16, 19, 20]. The value of 3D-CISS has been already demonstrated in hydrocephalus, and its advantage has been shown not only for demonstrating anatomical details but also for depicting the presence, location, and extension of membranes within the cisterns, which are of help for guiding ETV [16, 21]. Membranes in the foramen of Monro, superior velum medullaris, fourth ventricle outlets, and even intraventricular cystic masses may not visible in conventional T1- and T2-weighted images but are depicted with these sequences [16]. We routinely obtain sagittal 3D-CISS images in the midsagittal plane covering midline structures, basal cisterns, and fourth ventricle outlets with an extremely high resolution that allows reconstructions in any plane without losing data (Fig. 3.5).


Fig. 3.5
Sagittal 3D-CISS performed in the midsagittal plane in a 15-month-old patient with a fourth ventricle giant cyst, secondary hydrocephalus given an ETV. All the midline structures, ETV stoma, and basal cisterns can be evaluated in this sequence
3.2.4.3 Cine Phase Contrast
Cardiac-gated cine phase-contrast (PC) MRI is the only technique currently available to noninvasively detect CSF flow. It is a rapid, simple, and noninvasive technique that is sensitive to CSF flow [8, 15, 22, 23]. The PC-MRI generates signal contrast between flowing and stationary nuclei by sensitizing the phase of the transverse magnetization to the velocity of motion [24]. The technique provides “to-and-fro” CSF flow velocity and direction during a single cardiac cycle with a specially designed flow-sensitive GRE sequence. CSF flow is pulsatile and synchronous with the cardiac cycle; therefore, cardiac gating can be used to provide increased sensitivity [24, 25]. The cardiac-gated phase-sensitive technique is based on the subtraction of two similar GRE images, one with flow-encoding activated gradients and another with identical parameters but without these activated gradients. The difference in phase between the subtracted images is due to motion along the particular gradient axis. In this way, the signal from the stationary tissues is almost completely eliminated. Two series of PC imaging techniques are applied in the evaluation of CSF flow, one in the axial plane, with through-plane velocity encoding in craniocaudal direction for flow quantification, and another in the sagittal plane with in-plane velocity encoding in the craniocaudal direction for qualitative assessment [24].
The sensitivity of the flow-encoding gradients must be prospectively set to prevent aliasing. Qualitative midline sagittal and quantitative axial images perpendicular to the imaging plane, mostly the aqueduct, must be acquired. It takes a maximum of 10 min to get both sequences with gating [15, 16]. Flow in craniocaudal direction is encoded in shades of white, while flow in caudocranial direction is encoded in shades of black.
CSF flow MRI can be used to distinguish communicating and non-communicating hydrocephalus, to localize the level of obstruction in obstructive hydrocephalus, to detect communication of arachnoid cysts with the subarachnoid space, to differentiate between arachnoid pouches and subarachnoid space, to discriminate between syringomyelia and cystic myelomalacia, and to evaluate flow patterns in posterior fossa cystic malformations. This imaging method can also provide significant information in the preoperative evaluation of the Chiari 1 anomaly and of normal pressure hydrocephalus (NPH) and also in the postoperative follow-up of patients given an ETV or a VP shunt [24].
But cine PC has several disadvantages. To prevent aliasing artifact, which could affect the quality of both qualitative and quantitative assessments of cine PC, the strength of the velocity-encoding gradient needs to be set properly before scanning. On the other hand, cine PC demonstrates only bidirectional flow in a selected direction [16]. Whereas cine PC shows CSF flow, it is unable to demonstrate cisternal anatomic details and should be used in conjunction with 3D-CISS. Furthermore, this technique is limited because of poor visualization of turbulent flow and of its inability to measure bulk flow [8, 23].
3.2.5 Radionuclide Shunt Studies
Radionuclide CSF shunt studies can evaluate shunt patency and differentiate proximal versus distal shunt obstruction, and, in some cases, it may show the site of obstruction by indicating where isotopic activity fails to progress. In fact, the combination of CT and radionuclide imaging is more sensitive than CT alone in the diagnosis of shunt malfunction. The technique consists of injecting 99 mTc-pentetate or pertechnetate (0.25–1.5 mCi) into the valve reservoir with the patient supine. Care must be taken to ensure that the radiopharmaceutical is suitable for intrathecal injection because the sensitivity to endotoxin effects is higher for intrathecal injections than for the intravenous route. Immediately after the injection, dynamic imaging is performed for up to 30 min, typically using 30 s per frame. Activity progressively accumulates along the shunt tract and disperses quickly at the distal site of drainage. Radionuclide may also reflux into the ventricular system in certain models of valve. When the activity remains localized at the injection site, it indicates either obstruction or isotope extravasation. The latter is excluded by imaging the chest and abdomen for evidence of systemic absorption [4].
3.3 Normal Imaging Appearance of CSF Shunts
3.3.1 Ventricular Shunts
Hydrocephalus can be treated by CSF diversion or by endoscopic third ETV [26]. Ventricular shunting represents the traditional treatment for hydrocephalus. The anatomy of a ventricular shunt consists of three parts: the proximal catheter, the valve (with or without a reservoir), and the distal catheter. The catheters are typically made of silicone and have a diameter of 2–3 mm. Catheters are hypointense on T1- and T2-weighted MRI images and hyperdense on CT. The reservoir and valve are normally radiolucent, but they have radiopaque markers to allow for visualization (Fig. 3.1). The tip of the proximal catheter is placed at the widest part of frontal horn of the lateral ventricle, away from the choroid plexus and in front of the foramen of Monro [5]. The proximal catheter may be inserted either into the frontal or occipital regions through a small burr hole in the skull [26]. The catheter exits via the burr hole and connects to a reservoir that allows CSF fluid sampling and intraventricular pressure measurements. The reservoir is connected to the inflow port of a one-way valve, while the distal catheter is joined to the outflow port of the valve and is tunneled subcutaneously to end in the drainage cavity. The valve regulates the amount of CSF drainage and plays an essential role in the successful treatment of hydrocephalus [5]. In programmable shunts, the valve contains magnets that enable transcutaneous adjustments of the valve’s opening pressure [2, 4]. Shunts are commonly placed in the peritoneum, right atrium, or pleural space. VP shunting is the preferred method for CSF shunting by most neurosurgeons due to fewer complications and to the relative ease of peritoneal access [27]. Intra-abdominal pathology, such as peritoneal adhesions or recurrent peritonitis, necessitates placement or relocation into an alternative drainage site [4]. VA shunts are generally chosen when VP shunting has already failed or is contraindicated. The right atrium may be accessed percutaneously via the facial, subclavian, or internal jugular vein [28], and proper placement of the distal VA catheter may be ensured by real-time transesophageal echocardiography. The primary disadvantage of VA shunts is their risk of serious intravascular complications. On the other hand, ventriculopleural shunts have been rarely used for long-term shunting because of the high incidence of hydrothorax. Some authors have reported using ventriculopleural shunts for temporary CSF drainage in cases of VP or VA shunt infection and of tumoral hydrocephalus [4].
Radiologists are usually asked to assess shunt function by determining the position of the shunt tubing and for estimating the ventricular size. Decrease in ventricular size after shunting depends on several factors among them the cause of hydrocephalus. A prompt reduction as shown by a 24-h after shunting CT is expected in uncomplicated cases. CT scan will depict a normal or slit-like appearance of the ventricles in 60–80 % of shunted children [5]. If the ventricular size remains unchanged after 1–3 days, the patency of the shunt system should be questioned [26].
3.3.2 Endoscopic Third Ventriculostomy
ETV is an increasingly popular treatment of hydrocephalus since it avoids many of shunt-related complications, especially those of shunt dependence and overdrainage [21]. The main indication for performing an ETV is obstructive hydrocephalus, for example, in cases of aqueduct stenosis or tumors at its vicinity [26]. Age is the main determinant of outcome after ETV, with younger children, especially neonates, having a poorer prognosis [29]. Specifically, ETV resolves hydrocephalus in 80 % of children older than 2 years of age, but is not as effective in children younger than 2 years due to immaturity of the arachnoid granulations [21, 30, 31]. In ETV, an endoscope is used to create a hole in the floor of the third ventricle, immediately anterior to the mammillary bodies. It allows the passage of intraventricular CSF to the interpeduncular and suprasellar cisterns, from where it can flow laterally and upwards over the cerebral convexities to sites of CSF resorption [26]. If ETV does not result in adequate resolution of hydrocephalus, a ventricular shunt can still be placed.
Endoscopic management must also be considered before shunt replacement in cases of CSF shunt malfunction to avoid shunt-related complications. Some cases require staged endoscopic procedures to adequately communicate cavities in loculated hydrocephalus [32]. Decrease in ventricular size following ETV, in contrast with shunting, may be more gradual and occurs over several weeks [31]. MR is the study of choice for the assessment of the success of an ETV (Fig. 3.5) as only MR can assess the patency of the surgically created stoma in the floor of the third ventricle and observe the CSF pulsating through it [26].
3.4 Postoperative Complications After Shunt Placement (Table 3.1)
Table 3.1
Postoperative complications after CSF shunting
Complications |
---|
Intraventricular hemorrhage |
Parenchymal hemorrhage |
Subdural hematomas or hygromas |
Pneumocephalus |
Ventricular collapse/mantle inversion |
Subarachnoid hemorrhage |
3.4.1 Intraventricular Hemorrhage
Intraventricular hemorrhage during shunt revision in shunted patients is a potentially serious complication due to the almost constant adhesions of the proximal catheter tip to the choroid plexus and/or to ventricular walls [5, 33, 40]. Hemorrhage complicating shunt failure is a known predictor of future ETV failure. Intraventricular bleeding arises from subependymal vessels or from the choroid plexus when these vascular structures are injured by the ventriculoscope or by the endoscopic instruments. Generally, the blood loss is stopped by means of a continuous irrigation with Ringer’s solution to clear the clots or even one has to resort to placing an EVD if the hemorrhage continues [5, 33–35]. Postoperative neuroimaging may demonstrate a subependymal hematoma (Fig. 3.6) that might require an adjunctive surgical procedure for its management [33, 36].




Fig. 3.6
Postsurgical complications. (a) Axial CT with intraventricular hemorrhage in occipital horns after catheter placement. (b) Non-contrast cranial CT with intraventricular hemorrhage and clot around intraventricular catheter. (c) Postsurgical CT that shows parenchymal hemorrhage in right frontal lobe adjacent to the lateral ventricle. (d) CT in a patient with loculated hydrocephalus and pneumocephalus in temporal horn after shunt placement. (e) b1500 DWI MRI performed after urgent catheter replacement illustrating subacute ischemic infarction in left occipital lobe. (f) Axial cranial CT after ETV procedure with hematoma in the anterior limb of internal capsule and pneumoventriculus in the right frontal horn
The reported incidence of intraventricular hemorrhage ranges around 1–3 % of the cases [34, 37], although some authors indicate a higher incidence ranging between 3.5 and 6 % in infants [38, 39]. This higher rate could be explained in part by taking into account the less favorable ventricular anatomy in this age group, such as smaller Monro’s foramina and larger interthalamic adhesions, which would increase the risk of surgical damage during the surgical procedure [33].
Hemorrhages within the ventricles, which many times evolve in an urgent scenery, are usually assessed by CT.
3.4.2 Parenchymal Hemorrhage
The occurrence of intracerebral hematoma due to damage to the brain vessels during the introduction of the proximal catheter or the ventriculoscope, although possible, is rather uncommon but possesses an important potential morbidity [5, 33, 35, 41]. Large intraparenchymal and intraventricular hemorrhages are disastrous complications, especially in patients who have coagulopathies or are receiving anticoagulants (Fig. 3.6) [2]. These hemorrhages are well delineated in CT studies, and the smallest one does not require further surgical treatment.
3.4.3 Subdural Hematomas or Hygromas
Subdural hematomas or hygromas usually follow a benign course [5, 42]. In acute subdural hematomas, excessive loss of CSF during the endoscopic procedure is probably the main pathogenetic mechanism [33]. Post-shunting subdural collections have been reported to be more frequent in patients with long-standing macrocrania and subsequent craniocerebral disproportion characterized by a remarkably decreased intracranial compliance [33, 43]. On the contrary, subdural fluid collections after EVT have been only occasionally described (an overall rate of 0.5–1.5 % was reported in a large series) [35].
Neuroimaging studies (CT or MRI) contribute to the diagnosis of these subdural collections and are used to follow-up their evolution and to monitor the results of treatments. In general, the appearance of these collections does not differ from the normal images of subdural hygromas and hematomas found in other conditions.
3.4.4 Pneumocephalus
The presence of intracranial air following CSF shunting or endoscopic procedures is usually unimportant. However, tension pneumocephalus represents infrequent but dangerous complication [44]. Intracranial air (Fig. 3.6) results from the reduction in volume of the brain due to perioperative CSF leaks during the extrathecal CSF shunt implant or during the procedure of ETV [33, 45]. The presence of pneumocephalus, its volume, and distribution is clearly depicted with the current neuroimaging procedures (CT, MRI, and even with plain skull radiographs).
3.4.5 Other Complications
Another extremely rare complication of CSF overdrainage is the appearance of ventricular collapse and associated cerebral mantle inversion that are clearly depicted by CT or MRI studies [96]. They may require shunt replacement with flow-regulated valves, valve pressure upgrading, or addition of siphoning retarding [5]. Another unusual complication of CSF shunting is the occurrence of subarachnoid hemorrhage that is also well delineated by CT or MRI studies.
3.5 Intracranial Complications of CSF Shunts (Table 3.2)
Table 3.2
Intracranial complications of CSF shunts
Type of intracranial complication |
---|
Acute–subacute complications |
Shunt infection |
Obstruction |
Disconnection/fracture |
Catheter malpositions/migrations |
Overdrainage and slit ventricle syndrome |
Loculations |
Subcutaneous CSF collections and CSF fistula |
Complications of ETV |
Chronic complications |
Craniosynostosis |
Cranioencephalic disproportion |
Meningeal fibrosis |
Perishunt porencephaly and periventricular leukomalacia |
Trapped fourth ventricle |
Pneumocephalus |
3.5.1 Acute–Subacute Complications
3.5.1.1 Shunt Infection
Infection is a common etiology of shunt failure and represents the second cause of shunt dysfunction after mechanical malfunction [5, 33]. The reported incidence of shunt infection ranges from 1 to 40 % and average 8.5–15 % [33, 46, 47]. The majority of shunt infections occur in the postoperative period (70 % within 1 month, 85 % within 9 months) and are thought to be mainly due to contamination during the surgery [5, 27, 33, 50]. Intraoperative contamination by skin flora is the primary mode of infection, while other sources of shunt infection are proximal seeding from meningitis and distal seeding from peritonitis and wound infections [5]. Skin bacteria, such as S. epidermidis (50–90 % of the cases) and S. aureus (15–40 %), are most commonly found in early infectious complications [33, 51]. Other causative agents are gram-negative rods (15 %) and Propionibacterium acnes [5]. Shunt contamination may primarily originate from the skin incision proper and has been related to insufficient asepsis, defective surgical material, and long-lasting operations [33, 52].
Late infections are less common. In fact, only 10–15 % of overall infectious complications occur 1 year after the shunt implantation and are attributed to contamination of the distal catheter by the visceral content or infiltration of germs through superficial skin wounds [33, 50]. The involved pathogens in late infection (P. acnes, Enterococcus faecalis, Streptococcus faecalis) arise from the hair follicles or from the colonic contents and are capable of contaminating the catheter and even of reaching the cerebral ventricles [33, 50].
Infection in VP shunts may be evidenced by wound infection, fever, shunt malfunction, or peritonitis. VA shunts are associated with a similar incidence of infection; however, endocarditis and septic emboli result in higher morbidity and mortality. Similarly, infection of ventriculopleural shunts may result in empyema [4].
In comparison with extrathecal CSF shunts, infections in the context of ETV are not so problematic. The absence of the foreign body represented by the shunt apparatus limits the infection rate to 1–5 % of the cases [33–35, 48]. The incidence of infection seems to depend more on a history of previously infected shunts or external drainages than on the actual ETV procedure [33, 35, 37, 49].
The clinical signs of shunt infection are nausea, headache, and lethargy. Fever may or may not be present. Meningeal symptoms may also be absent because there is little communication between the infected ventricles and meningeal CSF. Lumbar and ventricular punctures are routinely performed to isolate these bacteria, but cultures are usually sterile. Instead, CSF obtained from the shunt reservoir typically allows for isolation of the causative organism [5]. Regarding management of shunt infection, several variants of treatments may be utilized [2, 5]. These will be discussed in detail in the corresponding chapter or the book.
CT and MRI may show irregular leptomeningeal and ventricular ependymal enhancement consistent with meningitis and ventriculitis, respectively [4]. Ventriculitis may be seen on contrast-enhanced CT or MRI as an irregular enhancement of the ependymal lining of the ventricles or of the cerebral cortical sulci [2, 5]. Debris within the ventricles, especially on diffusion-weighted imaging, are shown by differences in intensity between infected and normal fluid constituting the best neuroimaging sign of ventriculitis. Contrast-enhanced sequences demonstrate ependymal lineal contrast uptake. Pachymeningeal enhancement not due to infection can also be seen due to overshunting that can persist postoperatively for months [4, 5].
3.5.1.2 Shunt Obstruction
A shunt can be occluded at three points: the proximal catheter, the valve, and the distal catheter. The two most likely sites of obstruction are the ventricular catheter tip, which can be blocked by in-growing choroid plexus, and the shunt valve, which can be blocked by blood and debris [5].
In general, the postoperative period has the highest risk for shunt obstruction originated by debris and blood products. However, obstruction can occur at any time after shunt placement approaching a rate of 0.5 % per month [4, 5]. Ventricular catheter obstruction was found as the main cause of mechanical failure (63.2 %) followed by distal catheter occlusion (23.5 %), migration (8.8 %), disconnection (1.4 %), and breaking (1.4 %) [33].
Proximal catheter block accounts for 50 % of all failures within the first 2 years after shunt placement [4]. Proximal catheter obstruction frequently results from choroidal and ependymal reactions around the tip of the ventricular catheter. Actually, the proximity of the choroid plexus and the catheter tip is recognized as the most common cause of proximal shunt malfunction [33].
Whatever the site of obstruction, the symptoms are related to the secondary pathological increase in ICP [33]. The clinical presentation of shunt obstruction varies with age. Infants often present with nausea, vomiting, and irritability. Older children and adults, however, present with symptoms such as headache, nausea, vomiting, cranial nerve palsies, and ataxia [5, 27].
Imaging can reveal the position of the proximal catheter, normal connections, and ventricular enlargement (Fig. 3.7). Comparison of the last scan with preexisting or baseline studies is critical for demonstrating signs of either overt or subtle ventricular enlargement. When ventricular size is equivocal, blurring of the margins of the ventricles due to transependymal spread of CSF, perishunt edema, and subgaleal fluid collections suggests the presence of acute obstruction [5]. The proximal catheter can also migrate within the ventricle causing faulty CSF drainage. Outer migration of the proximal catheter is most commonly caused by traction of the distal catheter by scar tissue along the chest wall or the peritoneal entry site [27]. Diagnosis of shunt block is aided by comparing postoperative catheter placement on CT and conventional radiographs with images obtained when the patient becomes symptomatic [4].


Fig. 3.7
A 20-year-old-man given a VP shunt who presented with altered mental status. (a) Transaxial CT depicting diffuse hydrocephalus and transependymal CSF by distal shunt obstruction. (b, c) Abdominal US showing intraperitoneal fluid collection surrounding catheter tip. (d) Transaxial CT image and (e) sagittal reconstruction revealing intraperitoneal fluid collection surrounding the catheter tip in the left paracolic gutter. (f) Transaxial CT image 1 month later that demonstrates improvement in the left pseudocyst
3.5.1.3 Disconnection and Breakage
Disconnections most commonly occur shortly after shunt placement [2, 4]. They represent the second most common cause of shunt failure in children and most often occur in the neck that constitutes an area with greater mobility [2, 5]. Detachment of shunt components can occur at the connection of the shunt tubing to the reservoir or at the level of Y-shaped connectors, which are sometimes used to drain two ventricular cavities simultaneously (Fig. 3.8) [2]. Shunt disconnections became rare after the introduction of the one-piece or soldered shunts. Nowadays, disconnection is usually due to the stretching of junctions of the proximal or distal catheter to the valve or to fracture of the shunt tubing [33, 40].


Fig. 3.8
Distal catheter disconnection in a 10-year-old-patient with a Sophy valve and clinical suspicion of shunt malfunction. A disconnection of the distal catheter next to the valve is demonstrated in lateral cranial view of SS (a), sagittal (d), and coronal (e) 2D multiplanar reconstruction (MPR), 3D maximum intensity projection (MIP), (c) and VR reconstruction of cranial CT (f). Axial plane of cranial CT shows slightly enlarged ventricles and intraventricular catheter (b)
Material failures and surgical errors are often primary causes of shunt detachment [27]. Factors predisposing to disconnection include shunt aging, restricted mobility, repetitive trauma, and the presence of catheter connections and valve junctions. As shunts age, they undergo calcification and biodegradation rendering them more susceptible to breakage. The fibrous tissue that develops along the tubing also anchors the catheter leading to disconnection or fracture as the child grows [5].
Neuroimaging diagnosis of shunt disconnection is mainly made by the “shunt series” (Fig. 3.9) [27]. This diagnostic method reveals radiolucent gaps between segments and, in occasions, zones of calcification. It is important to remember that certain shunt components may also be radiolucent and should not be mistaken for disconnection (a common diagnostic pitfall). Comparison of these images with previous radiographs is mandatory as many shunting devices have sections of radiolucent tubing or valves [2, 4, 5]. Small collections of CSF can develop at the site of rupture or disconnection and are visualized on CT slices. CT can demonstrate subgaleal collections and enlarged ventricles too [2, 5, 33].


Fig. 3.9
Disconnection and breakage in lateral cranial SS. (a) Intraventricular retained catheter; note the presence of another valve (Sophy). (b) Reservoir detachment. (c) Disconnection and migration (arrows) of the reservoir. (d) Valve fracture after a head trauma
3.5.1.4 Catheter Malposition and Migration
Malposition of the proximal or distal limbs of shunts can result in malfunction. In certain cases, however, malfunction may not occur if the lateral apertures of the catheter remain within the ventricle as occurs when the position of the shunt tip is unchanged respect to prior studies. Shunt migration can occur at the distal or proximal end of the shunt (Fig. 3.10). Once the catheter is fixed to the subcutaneous tissues by fibrous tissue, the continued growth of the child results in traction and catheter migration. The proximal catheter can migrate to a non-draining area, such as the choroid, or to an extraventricular location in the parenchyma [5].


Fig. 3.10
Catheter misplacements. (a, b) Cranial lateral views of SS that show excessive length of the ventricular catheter. (c) Postoperative skull X-ray film depicting pneumocephalus and kinking of the distal catheter just at the back of the valve. (d) Axial CT depicting a curved intraventricular catheter and surrounding porencephaly
Flexion–extension of the head facilitates upward movement of the peritoneal catheter. In addition, the positive abdominal pressure against the negative suction from the intraventricular pressure, the loss of subcutaneous tissue, and the use of shunts with spring coils [53] might also contribute to the proximal migration of the catheter inside the ventricles, the subarachnoid, and subdural space or into the subgaleal scalp tissue [33, 54].
3.5.1.5 Overdrainage and Slit Ventricle Syndrome
Placement of CSF ventricular shunts may be complicated by overdrainage in variable proportions [55]. Diverse manifestations of excessive CSF drainage occurred in approximately 18 % of hydrocephalic children in one of our series [56]. Ventricular size returns to normal within 24 h after shunt placement with a subsequent more gradual reduction that depends on the cause and chronicity of hydrocephalus. However, if the lateral ventricles collapse too rapidly, the brain may not be elastic enough to fill the space. The resulting disparity between the brain size and skull (Fig. 3.11) leads to formation of subdural hygromas or hematomas [4, 5].


Fig. 3.11
CT of overdrainage. (a) Intraventricular catheter with dysmorphic ventricles, bilateral subdural hygromas and sclerosis of the coronal suture. (b) Intraventricular catheter with frontal subacute subdural hematoma, right frontal arachnoid cyst, and cerebral collapse
Chronic overdrainage is relatively common and is shown as small-sized or slit-like ventricles on neuroimaging studies in up to 50 % of shunted children [4]. Slit-like ventricles are nonsignificant clinically in the majority of cases. The interest of this finding rests on the association of slit-like ventricles to its symptomatic counterpart called “slit ventricle syndrome” (SVS). This syndrome is defined as the presence of intermittent symptoms of raised ICP associated with the slit-like appearance of the ventricles on neuroimaging studies [33].
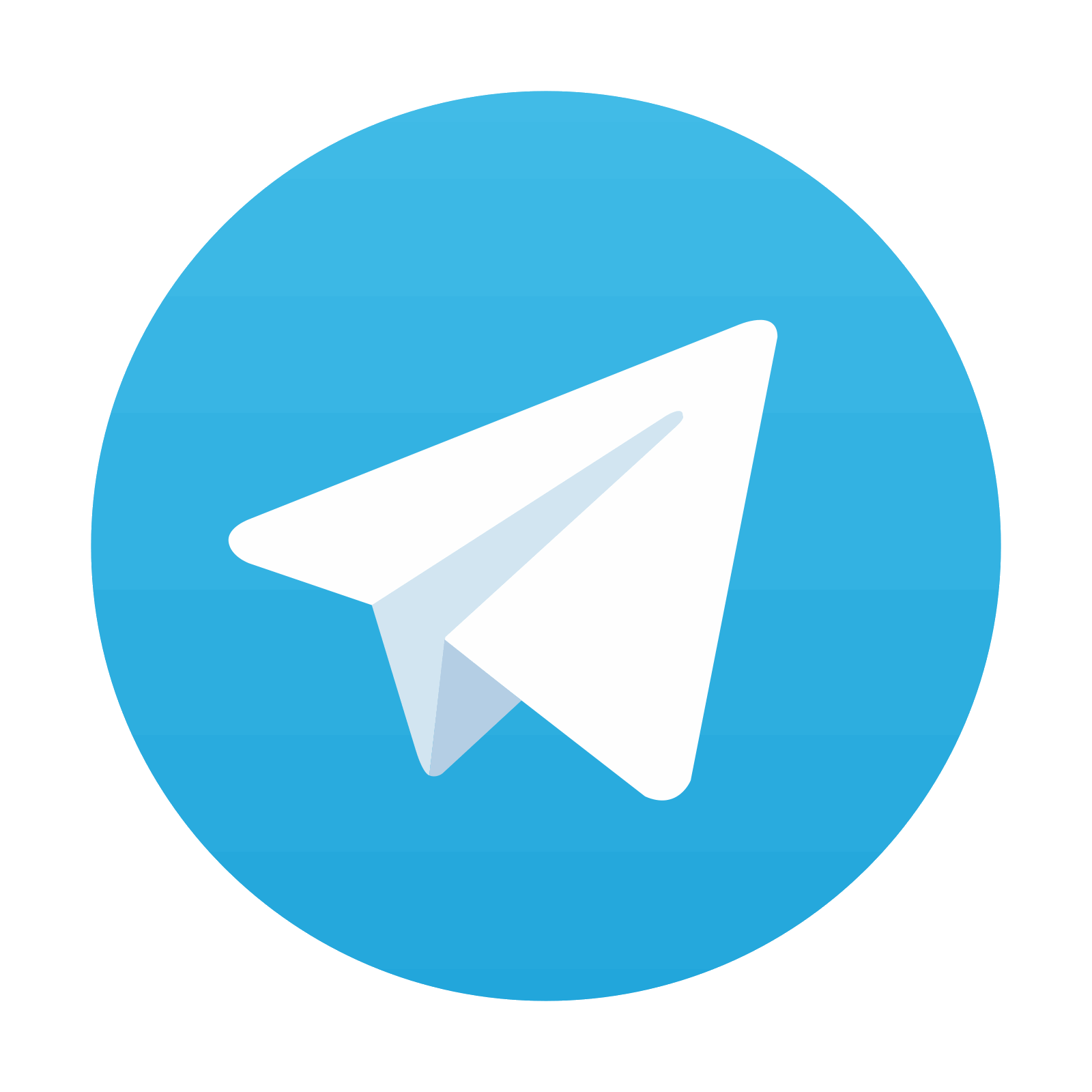
Stay updated, free articles. Join our Telegram channel
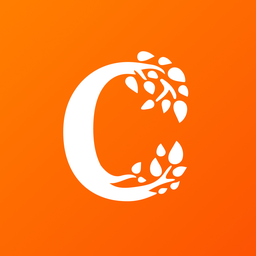
Full access? Get Clinical Tree
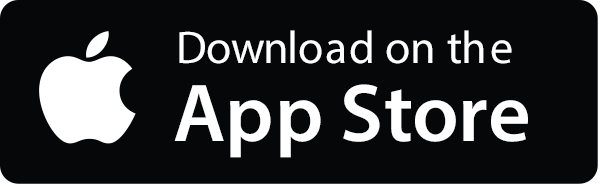
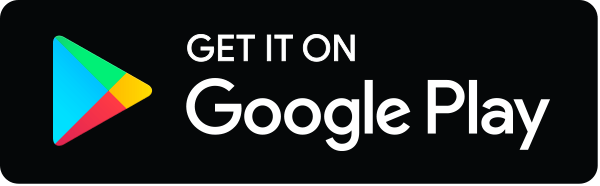