Advances in imaging technology have played a key role in the progress made over the last two decades in the treatment of patients with neurological disease, facilitating the identification, classification, and documentation of the different pathologic processes that constitute the subspecialty fields of neurology. As such, neuroimaging is an integral part of both the training and practice of this specialty, with many subspecialists spending a considerable portion of their time in the application of imaging techniques for the diagnosis and treatment of their patients.
The most practical approach to a discussion of the application of neuroimaging techniques in neurological care is to first address the tasks, diagnostic or therapeutic, that require their utilization. From this perspective, the clinical scenarios in which imaging techniques are likely to be needed must be assessed along the following lines:
- What information is being sought, and how quickly is it needed?
- Which of the available imaging techniques is most likely to answer the question being asked?
- How will the information obtained by imaging impact further diagnostic algorithms, the treatment, and the prognosis of the patient?
Based on these considerations, the tasks that require the utilization of imaging during clinical care include initial diagnosis, categorization and therapeutic allocation, imaging of the brain blood vessels, characterization of the pathologic process and its consequences (edema, mass effect, etc.), and assessment of prognosis.
Direct imaging of the brain is necessary in order to assess the status of the tissue. The techniques available to complete this task include computed tomography (CT) and magnetic resonance imaging (MRI). As it will be discussed below, choosing one or the other involves considerations of speed, sensitivity, type of lesion, resource availability, and temporal profile of the event.
Once the diagnosis is made, a more precise definition of the condition has enormous therapeutic implications, both immediate and long-term. The same technologic advances described above have extended to improvements in image resolution, with better signal-noise ratios, and a superior definition of the pathologic processes. Furthermore, since the cerebral vasculature is so often implicated in the pathogenic process, imaging of the cerebral arteries and veins becomes an additional dimension in the categorization of these patients.
Imaging of the brain blood vessels is an integral component of the evaluation of stroke, vascular anomalies and in some cases, tumor evaluation (especially in the surgical planning stage). In general, the tests that are available for the completion of this task are divided into two groups: (1) those that are non-invasive (ultrasonic, radiotomographic, and magnetic-based techniques) and (2) those that are invasive (catheterization and angiography). In addition to its diagnostic capabilities, the latter also allows the application of endovascular therapeutic techniques.
As discussed earlier, documentation of the structural abnormalities can be accomplished using CT and MRI. MRI can be utilized to further identify the brain connectivity (MRI diffusion tensor imaging), vascular perfusion (MRI perfusion-weighted imaging), and chemical activity (MRI spectroscopy). Nuclear-based imaging techniques, such as single photon emission computed tomography (SPECT) and positron emission tomography (PET), also provide mechanisms for functional imaging of the brain.
Following identification and categorization of the lesion, it is possible to use the imaging information to approximate the potential outlook and final outcome of each patient. Furthermore, it is often possible to make predictions about the potential benefit of a specific treatment strategy. Clearly, this characteristic of imaging relates to the fact that the existing techniques represent the equivalent of “bedside neuropathology.”
Every discussion about modern neuroimaging techniques must begin with computed tomography (CT). Its introduction in the 1970s changed the way all neurological diseases are diagnosed and treated. With CT, it became possible to directly look at the brain tissue, and document the damage from a particular lesion. The principles of CT are relatively simple: narrow beams of X-rays are used to rotationally acquire tissue density information in one of several tomographic planes. The raw data acquired over a period of a few minutes is then entered into a computer that reconstructs the tissue sample in two-dimensional tomographic images. At present, fast helical scanning techniques, and powerful reconstruction algorithms, allow even three-dimensional reconstruction of the brain tissue and the brain blood vessels. The images obtained represent a map of the density of tissue in a scale of Hounsfield units (HU, Figure 2-1) that spans between –1000 (the green corresponding to air) and +1000 (the white corresponding to calcium). CT is particularly useful in the urgent evaluation of stroke, trauma, monitoring edema, and the evaluation of calcifications.
Figure 2-1.

Relationship between the various tissues and their corresponding Hounsfield units (HU) in computed tomography (CT). Density increases as HU increases. Note that the HU (and the density of intracranial hemorrhage, ICH) is greater than white matter (WM) and of gray matter (GM). (CSF, cerebrospinal fluid.) (Reproduced with permission from Geyer J, Potts D, Dillard S, Gomez C. Neuroimaging in the management of stroke patients. In: Geyer J, Gomez CR, eds. Stroke: A Practical Approach. Philadelphia: Lippincott Williams & Wilkins; 2009:167.)
CT angiography is a relatively noninvasive technique that couples helical CT scanning with contrast enhancement to obtain vascular images. It is performed by first obtaining a series of helical axial images following the injection of iodinated contrast. These are then reconstructed using 1.25-mm thick slices and 0.5- to 1.0-mm increments. This protocol allows three-dimensional rendering of the angiographic images (Figure 2-2). The development of this technique required the introduction of a scanner that allowed the patients to be translated through a continuously rotating gantry, with very rapid data acquisition. The operator controls several variables that determine the protocol to be utilized for each region of interest to be imaged, including duration of the scan, speed of movement of the table, and collimation. As with any other technique, CT angiography has advantages and disadvantages. On one hand, it is not susceptible to flow perturbations and complex flow patterns like magnetic angiography. On the other hand, it utilizes ionizing radiation and iodinated contrast administration, which limits its use in patients with azotemia and contrast allergy, while resulting in a more limited field of view (FOV) per study. The latter is very important, for it requires that the planning and post-processing of the study take into consideration the question being asked. Furthermore, post-processing and three-dimensional rendering typically involve “sculpting out” some of the tissue that surrounds the vessels, based on its density. This process, when not carefully carried out, can conceivably lead to the exclusion of important structures or pathologic findings. Its best application appears to be in the definition of intracranial aneurysms prior to their treatment (Figure 2-3).
Figure 2-2


Three-dimensional rendering of the intracranial arteries using computed tomographic angiography (CTA). The arteries are displayed by themselves in the axial plane (A). It is also possible to display them in the context of the surrounding bony environment (B). (Reproduced with permission from Geyer J, Potts D, Dillard S, Gomez C. Neuroimaging in the management of stroke patients. In: Geyer J, Gomez CR, eds. Stroke: A Practical Approach. Philadelphia: Lippincott Williams & Wilkins; 2009:168.)
Figure 2-3

Use of computed tomographic angiography (CTA) to diagnose aneurysms. Three-dimensional rendering of the CTA clearly identifies a left posterior communicating artery aneurysm (arrow). (Reproduced with permission from Geyer J, Potts D, Dillard S, Gomez C. Neuroimaging in the management of stroke patients. In: Geyer J, Gomez CR, eds. Stroke: A Practical Approach. Philadelphia: Lippincott Williams & Wilkins; 2009:168.)
The utilization of magnetic resonance imaging (MRI) in clinical medicine became widespread during the 1980s. The increased sensitivity of this technique, as compared with CT, for the detection of abnormalities in brain structure made it an immediate candidate for the imaging modality of choice. MRI has allowed the documentation of brain lesions earlier and more precisely, particularly in regions that had remained relatively poorly visualized by CT, such as the brainstem. More recently, the introduction of additional MRI sequences has resulted in the ability to image the brain vessels, as well as to study the chemical composition of the tissues being imaged and viability of the tissue.
MRI is particularly well suited to the identification of cerebral edema (increased water content, whether related to tumor, injury, or infarction). Increased tissue signal on T2-weighted, proton density, and fluid-attentuated inversion recovery (FLAIR) images, as well as a less pronounced drop in signal on T1-weighted sequences, accompanies this pathophysiologic process. These findings are evident in MRI of the chronic infarction shown in Figure 2-4. As opposed to the flow-related findings noted earlier, the parenchymal changes evolve over a period of hours to days.
Figure 2-4



Appearance of a chronic infarction of a large portion of the right middle cerebral artery on magnetic resonance imaging, using spin-echo (SE) sequences. T2-weighted imaging shows the entire area of infarction as hyperintense (A). Fluid-attenuated inversion recovery (FLAIR) images display a core of low intensity with a margin of high intensity (B). The cystic transformation of the damaged tissue can be greatly appreciated. T1-weighted images show the area as hypointense (C). (Reproduced with permission from Geyer J, Potts D, Dillard S, Gomez C. Neuroimaging in the management of stroke patients. In: Geyer J, Gomez CR, eds. Stroke: A Practical Approach. Philadelphia: Lippincott Williams & Wilkins; 2009:169.)
The MRI appearance of intracranial hemorrhages over time is one of the most interesting subjects in neuroimaging, for it largely depends upon the natural evolution of hemoglobin degradation within the tissue and the strength of the magnetic field (Figure 2-5). Typically, the sequence of conversion from oxyhemoglobin to deoxyhemoglobin, followed by that from deoxyhemoglobin to methemoglobin (first intracellular and then extracellular, as the erythrocytes disappear), and finally to hemosiderin, occur as parts of a continuum that evolves over weeks to months.1 Hyperacute hemorrhage (ie, a few hours), in the form the oxyhemoglobin, is isointense with the brain parenchyma on T1-weighted spin echo (SE) images, and hyperintense on T2-weighted SE images. After a few hours, the oxyhemoglobin evolves into deoxyhemoglobin within the hematoma (Figure 2-5). The latter predominantly shortens T2, and this leads to low signal on T2-weighted images. After 3 to 4 days, deoxyhemoglobin is progressively converted to methemoglobin, which is a paramagnetic substance that shortens both T1 and T2, although its predominant effect is shortening T1 (Figure 2-5). As a result, at this stage, hematomas display high signal in both T1- and T2-weighted images. Over the next few months, the methemoglobin is slowly broken down into hemichromes that produce only mild T1 shortening. Hematomas at this end stage have a slightly high signal on T1-weighted images, but retain a high signal on the T2-weighted images. Finally, around the periphery of hematomas, macrophage activity results in degradation of the methemoglobin and conversion of the iron moiety to hemosiderin, which shortens T2 and produces a black ring around the hematoma on T2-weighted images. This can be observed within 2 weeks after hemorrhage, and it has a tendency to become thicker over time. In small hematomas (less than 1 cm), the low signal intensity from hemosiderin may essentially occupy the entire ultimate volume of the cavity. The length of time that the hemosiderin will remain in the area of a hematoma mimics autopsy findings many years after an intracerebral hemorrhage, and it is suspected, using high-field MRI, the hemorrhage can be readily identified over the lifetime of the patient.
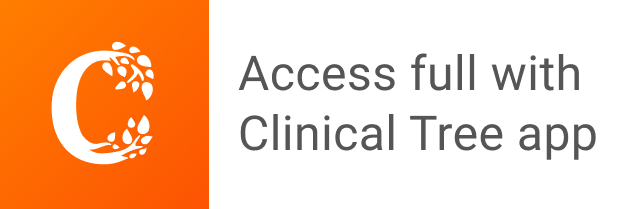