Fig. 8.1
Hypothalamus–pituitary–adrenal (HPA) axis response to peripheral LPS in mice prenatally exposed to VPA. Animals prenatally exposed to VPA show a normal HPA response postnatally, but an exacerbated response in adulthood. Plasma corticosterone levels were measured 2 h after a 25 mg/kg LPS i.p. injection. Values are expressed as a percentage with respect to animals prenatally exposed to saline and injected 2 h before with saline. LPS lipopolysaccharide, VPA valproic acid
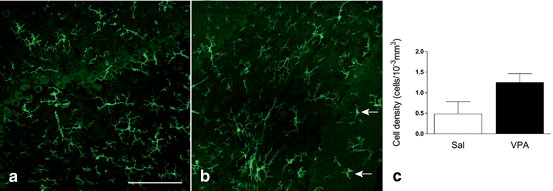
Fig. 8.2
Activated microglia in the postnatal cerebellar lobe 7 of VPA-exposed mice. Confocal microscopy photographies after IBA1 immunofluorescence of cerebellar tissue obtained at P21 from saline (a) and VPA (b) mice. The arrows point type II–III microglial cells, mainly observed in animals prenatally exposed to VPA. c Type II–III microglial cell density in the molecular layer of the cerebellar lobe 7. VPA valproic acid
In addition, mice exposed prenatally to 500 mg/kg VPA showed intestinal inflammation at PD28 (de Theije et al. 2013) . VPA mice showed loss of the epithelial barrier and neutrophilic infiltration in the ileum. These results give support to the hypothesis of an association between the GI diseases frequently observed in autistic individuals and their behavioral symptoms.
Although we still cannot distinguish between VPA causing the immune and behavioral alterations by two independent mechanisms or inflammation mediating the effects of VPA on behavior, the reports previously cited brought into light a valuable tool for these studies.
8.3.3 Inflammation and Immune Alterations in Genetic Models of Autism
Autism is a clinically heterogeneous disorder that in some cases can be associated with genetic disorders such as Rett and fragile X syndrome, or tuberous sclerosis. However, in the great majority of the patients a unique pathophysiological mechanism has not been able to be identified and the causes of the disorder remain unknown (Pardo and Eberhart 2007) .
As diverse evidences suggest the existence of a heritable component in ASD, numerous animal models have been generated with the manipulation of candidate genes identified by means of population studies. Some mutant lines are based on monogenic aberrations and others on loci that confer susceptibility to the disease. Nevertheless, the attempts to link individual mutations with ASD have failed (Wassink et al. 2004) , and nowadays there is consensus about the existence of a multiplicity of genes that confer susceptibility to the disease, which are influenced both by environmental factors and by their interactions with other genes.
In a study carried out by Moy et al. (2007) , male mice from ten inbred strains were characterized for several autism-related behaviors such as sociability, preference for social novelty, and reversal learning of the spatial location of a reinforcer in two different mazes. Interestingly, they found that the BTBR T + tf/J strain displays reduced sociability and resistance to change in routine in the water maze. As these findings are consistent with an ASD phenotype, some groups have proposed to use the BTBR strain as a model of autism .
Other groups further characterized the autism-related behaviors in the BTBR strain (McFarlane et al. 2008; Amodeo et al. 2012; Pearson et al. 2011, 2012; Pobbe et al. 2010) . They found that BTBR mice show reduced social approach, low reciprocal social interactions, impaired juvenile play, and absence of social conditioned place preference. Moreover, they display high levels of self-grooming and impaired social transmission of food preference as compared with C57BL/6J mice, and resistance to reversal learning. In addition, BTBR mice also show an unusual repertoire of USVs both as pups and as adults (Scattoni et al. 2008, 2011; Wohr et al. 2011) .
These results show that BTBR mice display the three core features of autism: reduced sociability, repetitive behaviors, and impaired communication. The next step was to characterize the immune system of these mice, which was conducted by Heo et al. (2011) . In this study, levels of brain-reactive antibodies were higher in BTBR serum and brains, and they also presented elevated brain expression levels of pro-inflammatory cytokines such as IL-33, IL-18, and IL-1β. Additionally, BTBR mice presented higher susceptibility to listeriosis infection.
As the ability of macrophages to switch to an inflammatory profile is known to confer immunity to listeriosis, another study hypothesized an association between myeloid inflammation and ASD behavioral phenotype. Onore et al. (2013) aimed to examine the role of macrophage polarization (M1/M2) in the BTBR strain. BM macrophages from BTBR mice present higher inflammatory cytokine production with or without LPS stimulation. When M1 or M2 phenotypes were induced by the addition of polarizing cytokines to the media, BTBR macrophages exhibited a trend towards an M1 phenotype. They also evaluated the relationship between the behavioral and immune phenotypes by measuring social and repetitive behavior in each individual mouse and analyzing its correlation with the cytokine profile. The experiments showed that grooming behavior was associated with several cytokines, suggesting the existence of a direct relation between inflammatory cytokines and more impaired repetitive behavior on one hand, and of anti-inflammatory cytokines and behavioral improvement on the other. Conversely, they found very little association between social behavior and cytokine expression profiles, indicating that sociability may not be strongly affected by these cytokine alterations. This study suggests that the behavioral phenotype and the inflammatory immune profile of BTBR mice are not independent, but a connected phenomena.
8.3.4 Combining Genetic and Inflammatory Models
As it is now believed that ASD is not a consequence of a unique pathophysiological cause but the result of combining environmental, genetic, and epigenetic factors; it is important to study how these factors interact between themselves.
In line with this, Schwartzer et al. (2013) hypothesized that the genetic background of mice could influence the severity of the ASD phenotype elicited by an environmental risk factor such as the MIA. To test this hypothesis, BTBR and C57BL/6J (C57) pregnant dams were injected with polyI:C on GD12.5 and their offspring were tested for ASD-like behaviors. Both in BTBR mice as in C57 controls, maternal polyI:C injection altered USVs, impaired social behavior, and increased repetitive behaviors in the offspring. However, some of these effects were stronger on BTBR mice.
Interestingly, they also evaluated the induced response of splenocytes, measuring cytokine levels. Interestingly, they observed a significant strain x prenatal treatment interaction in the release of IL-17, with BTBR prenatally exposed to polyI:C secreting the highest amount of this cytokine.
These results are in line with evidences obtained in MIA and BTBR autism models, which demonstrate that both environmental factors and genetic predisposition can result in similar behavioral ASD phenotypes. Moreover, this study adds evidence to the synergistic hypothesis, as the combination of genetic and environmental factors exacerbates the ASD phenotype generated by either factor alone. Further studies would help elucidating the mechanisms through which inflammation can contribute to autism-related behaviors in both environmental and genetic models.
8.4 Conclusions
Animal models are very valuable tools to study the biological mechanisms underlying psychiatric disorders. Different groups have started using them for evaluating the role of immune alterations in the development of autism-related behaviors. Evidence shows that diverse immune stimuli can result in reduced sociability and increased repetitive behaviors. In addition, genetic and environmental models of autism showed immune dysfunction. Although the mechanisms are not elucidated, all these evidences suggest a role of immune factors in determining autism-related behavior and prove relevance to the studies with animal models of autism.
References
Abrahams BS, Geschwind DH (2008) Advances in autism genetics: on the threshold of a new neurobiology. Nat Rev Genet 9(5):341–355. doi:nrg2346 [pii] 10.1038/nrg2346PubMedCentralPubMedCrossRef
Adams JB, Johansen LJ, Powell LD, Quig D, Rubin RA (2011) Gastrointestinal flora and gastrointestinal status in children with autism–comparisons to typical children and correlation with autism severity. BMC Gastroenterol 11:22. doi:10.1186/1471-230X-11-22PubMedCentralPubMedCrossRef
Amodeo DA, Jones JH, Sweeney JA, Ragozzino ME (2012) Differences in BTBR T + tf/J and C57BL/6J mice on probabilistic reversal learning and stereotyped behaviors. Behav Brain Res 227(1):64–72. doi:10.1016/j.bbr.2011.10.032PubMedCentralPubMedCrossRef
Balemans MC, Huibers MM, Eikelenboom NW, Kuipers AJ, van Summeren RC, Pijpers MM, Tachibana M, Shinkai Y, van Bokhoven H, Van der Zee CE (2010) Reduced exploration, increased anxiety, and altered social behavior: Autistic-like features of euchromatin histone methyltransferase 1 heterozygous knockout mice. Behav Brain Res 208(1):47–55. doi:10.1016/j.bbr.2009.11.008PubMedCrossRef
Chen SK, Tvrdik P, Peden E, Cho S, Wu S, Spangrude G, Capecchi MR (2010) Hematopoietic origin of pathological grooming in Hoxb8 mutant mice. Cell 141(5):775–785. doi:S0092-8674(10)00374-0 [pii] 10.1016/j.cell.2010.03.055PubMedCentralPubMedCrossRef
Crawley JN (2007) Mouse behavioral assays relevant to the symptoms of autism. Brain Pathol 17(4):448–459. doi:BPA096 [pii] 10.1111/j.1750-3639.2007.00096.xPubMedCrossRef
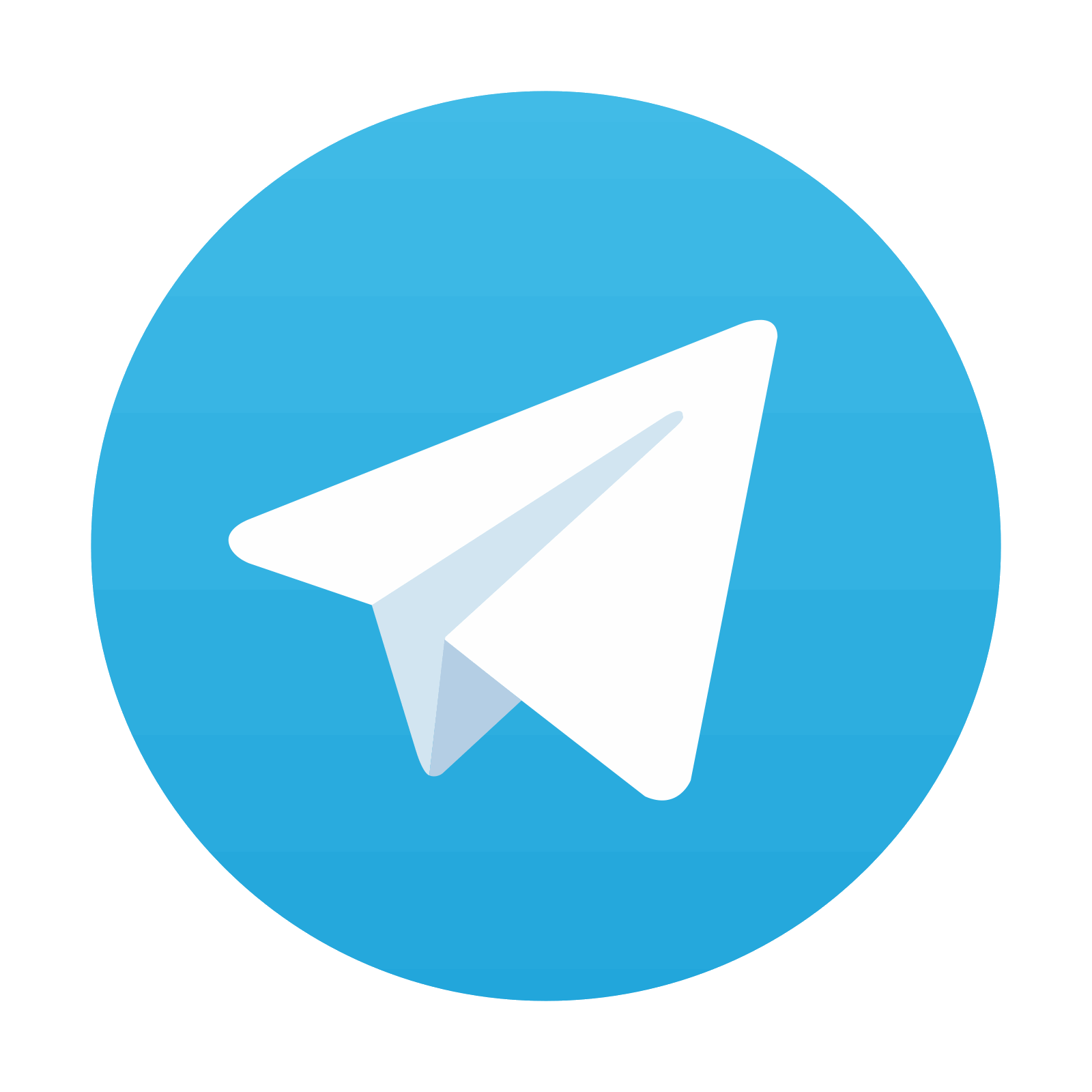
Stay updated, free articles. Join our Telegram channel
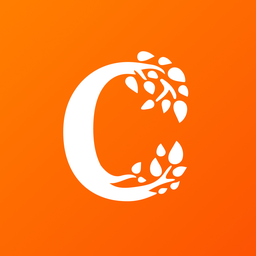
Full access? Get Clinical Tree
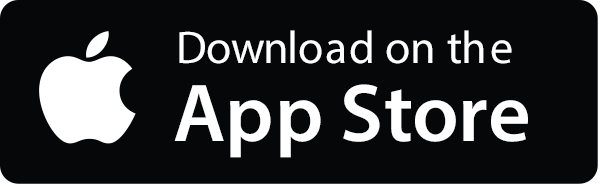
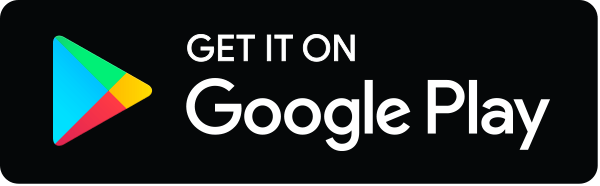