Introduction
Immune-based therapies have revolutionized cancer treatment. These agents enhance the immune system’s ability to seek out and attack tumor cells. One such treatment, CAR T-cells or chimeric antigen receptor T-cells, are a type of adoptive cell transfer therapy where a patient’s own T-cells are collected, genetically modified to treat their cancer, and reinfused. CAR T-cell therapy starts with collecting autologous T lymphocytes from a patient via leukapheresis. These cells are genetically engineered to express a modified T-cell receptor known as a chimeric antigen receptor (CAR), which binds to a specific cancer antigen such as CD19. Once CAR T-cells express the chimeric receptor of interest in vitro, they are expanded, purified, and infused back into the patient after the patient has received lymphodepleting chemotherapy. Once infused, cancer antigens stimulate the CAR T-cells to release cytotoxins, inducing cancer cell death, and resulting in treatment responses.
Structure, function, and administration of CAR T-cells
CARs are genetically engineered fusion proteins that redirect the specificity and function of T-cells. The CAR protein is composed of an extracellular antigen-recognition domain, a transmembrane domain, and an intracellular signaling module derived from T-cell signaling proteins. Each CAR contains the CD3ζ protein which plays a role in the activation of a T-cell.
- •
First-generation CARs contain CD3ζ
- •
Second-generation CARs possess a costimulatory endodomain (e.g., CD28 or 4-1BB) fused to CD3ζ
- •
Third-generation CARs consist of two costimulatory domains linked to CD3ζ
After a CAR construct is transfected into autologous or allogeneic peripheral blood T-cells using plasmid transfection, mRNA, or viral vector transduction, the T-cells are infused into the patient to target whichever surface-exposed tumor antigen is specified by the CAR’s extracellular targeting moiety, usually in the form of a single-chain variable fragment. Upon CAR engagement of its associated antigen, primary T-cell activation occurs and leads to cytokine release, cytolytic degranulation, and T-cell proliferation. Unlike vaccines and immunomodulatory agents that rely on in vivo priming of endogenous tumor-reactive cells and are therefore human leukocyte-antigen (HLA) restricted, CAR T-cells are capable of inducing durable antitumor responses in a universal, HLA-independent manner.
Leukapheresis . Leukapheresis is the first step in developing CAR T-cells for use in an individual patient. First, blood is removed from the patient, leukocytes are separated, and the remainder of the blood is returned to the circulation. After a sufficient number of leukocytes have been harvested, the leukapheresis product is enriched for T-cells. Next, the T-cells undergo an activation process during which they are incubated with the viral vector encoding the CAR, and after several days, the vector is washed out of the culture. Lentiviral vectors are used most frequently, but other methods of gene transfer are being explored. , When the cell expansion process is finished, the cell culture is concentrated to a volume that can be infused into the patient and is cryopreserved in infusible medium. Finally, when the product is released for treatment, the frozen cells are transported to the treatment site and thawed prior to administration. In patients with hematologic malignancies, lymphodepleting chemotherapy is typically administered prior to the CAR T-cell reinfusion. Lymphodepletion can substantially increase the in vivo expansion of the infused CAR T-cells by multiple effects, including reducing the patient’s lymphoid cell pool to make “space” for the CAR T-cells, increasing homeostatic cytokines, and ameliorating the tumor inhibitory microenvironment.
Efficacy and toxicity of CAR T-cell therapy
Efficacy. The greatest advances for CAR T-cells have occurred in the treatment of hematologic malignancies, with the United States Food and Drug Administration (FDA) having approved two therapies as of the writing of this chapter. Tisagenlecleucel, a CD19-targeted CAR T-cell therapy formerly known as CTL019, was first approved for the treatment of patients up to 25 years of age with B-cell precursor acute lymphoblastic leukemia (ALL) that is refractory or in second or later relapse. Tisagenlecleucel was subsequently approved for adult patients with relapsed or refractory large B-cell lymphoma after two or more lines of systemic therapy, including diffuse large B-cell lymphoma (DLBCL), high-grade B-cell lymphoma, and DLBCL arising from follicular lymphoma. The second CAR T-cell therapy approved by the FDA was axicabtagene ciloleucel, which is also targeted against CD19 and is approved for adult patients with relapsed or refractory large B-cell lymphoma patients who have failed at least two prior therapies, including DLBCL, primary mediastinal large B-cell lymphoma, high-grade B-cell lymphoma, and DLBCL arising from follicular lymphoma. Remarkably, both of these CAR products have led to durable remissions in patients with B-cell malignancies refractory to standard salvage therapies, with an overall response rate of 50–90% across multiple trials.
Cytokine release syndrome. CAR T-cell therapies for hematologic malignancies have unique toxicities that are distinct from those of cytotoxic chemotherapy, small-molecule targeted therapies, and even from other immunotherapies. The most commonly observed toxicity with CAR T-cells is cytokine-release syndrome (CRS), which manifests as high fever, hypotension, hypoxia, and/or multiorgan toxicity. Rarely, cases of CRS progress to fulminant hemophagocytic lymphohistiocytosis (also known as macrophage-activation syndrome), which is characterized by severe immune activation, lymphohistiocytic tissue infiltration, and immune-mediated multisystem organ failure. , CRS is triggered by the activation of T-cells upon engagement of their CARs with cognate antigens expressed by tumor cells. When this occurs, both the activated T-cells, as well as bystander immune cells including monocytes and macrophages, release cytokines and chemokines that lead to a systemic inflammatory state that resembles sepsis physiology. CRS usually manifests with constitutional symptoms, such as fever, malaise, anorexia, and myalgias, but can ultimately impact any organ in the body, including the heart, lungs, gut, liver, kidneys, bone marrow, or nervous system. CRS should be managed in accordance with the grade of this toxicity, which can be determined using established guidelines. Detailed discussion of the management of CRS is beyond the scope of this chapter, but interventions typically include supportive care such as intravenous fluid boluses, anti-interleukin (IL)-6 therapy with tocilizumab or siltuximab, and/or corticosteroids in cases refractory to anti-IL6 therapy.
In addition to ALL and DLBCL, CAR T-cells have shown promise in early phase trials in other hematologic malignancies, including multiple myeloma. , In solid tumors, however, response rates have been much less favorable. Some of the most significant challenges for CAR T-cell immunotherapy in solid cancers include (1) identification of unique tumor target antigens, (2) improved CAR T-cell trafficking to tumor sites, (3) addressing tumor heterogeneity and antigen loss, and (4) manipulating the immunosuppressive tumor microenvironment to allow for effective CAR T-cell expansion and tumor cell killing. While improved efficacy of CAR T-cells in solid tumors is eagerly awaited, patients enrolled on solid tumor CAR T-cell trials are at risk of many of the same complications as patients with hematologic malignancies. Thus, it is imperative that CRS and other compilations of CAR T-cells are recognized and managed appropriately by neurologists and other members of the care team. The remainder of this chapter will focus specifically on the neurotoxicity associated with CAR T-cell therapies.
Clinical case
Case . A 69-year-old male with a history of refractory DLBCL presented with progression of his tumor despite two recent cycles of salvage chemotherapy with R-ICE (rituximab, ifosfamide, carboplatin, and etoposide). He was recommended for CAR T-cell therapy with axicabtagene ciloleucel. T-cells were collected, and he was admitted to the hospital for CAR T-cell infusion. He received lymphodepleting chemotherapy with fludarabine and cyclophosphamide followed by infusion of CAR T-cells. He was initiated on seizure prophylaxis with levetiracetam 750 mg by mouth twice daily, which is standard. On day +1 following the infusion, he became febrile to 101.0°F. An infectious workup was initiated and was negative. On day +2, he developed an increasing oxygen requirement requiring a nonrebreather mask and was given a single dose of tocilizumab 8 mg/kg intravenously (IV) and dexamethasone 10 mg IV for presumed CRS. His hypoxia and fevers improved, but on day +5 following the infusion, he developed expressive aphasia, increased somnolence and confusion, and bowel/bladder incontinence. His somnolence and confusion progressed rapidly and he was transferred to the neurological intensive care unit. A non-contrast head CT was unremarkable and showed no evidence of intracranial hemorrhage or cerebral edema. MRI of the brain with and without contrast demonstrated diffuse, nonspecific T2-weighted and fluid-attenuated inversion recovery (FLAIR) signal abnormality in the periventricular and subcortical white matter. He was initiated on dexamethasone 10 mg IV every 6 hours. Despite this intervention, his mental status continued to decline, and he was intubated for airway protection. A lumbar puncture was performed. Cerebrospinal fluid (CSF) protein was elevated at 509 mg/dL, but other CSF studies were within normal limits. A 24-hour continuous electroencephalogram (EEG) was performed without any seizures or clinical events captured and demonstrated generalized slowing and sharp waves with triphasic morphology. He remained intubated for 2 days and was extubated on day +8 following CAR T-cell infusion when his mental status began to improve. He showed continued daily clinical improvement, and his corticosteroids were tapered rapidly. By day +20, his neurotoxicity had completely resolved, and by day +24, he was no longer on steroids.
Teaching Points . This case describes a typical presentation for a patient suffering from neurologic toxicity from CAR T-cell therapy. Neurotoxicity in the setting of CAR T-cell therapy has been referred to both as immune effector-cell associated neurotoxicity syndrome (ICANS) and CAR-related encephalopathy syndrome (CRES). Neurotoxicity is thought to develop either as a result of systemic cytokine release propagating into the central nervous system (CNS) or from direct migration of CAR-T-cells into the CNS. There is a bimodal distribution with neurotoxicity either developing early after CAR-T infusion usually in the setting of CRS or occurring several weeks after the infusion often without evidence of CRS. Nonspecific neurologic symptoms such as headache, malaise, nausea, and fatigue are not necessarily indicative of ICANS. However, red flag symptoms include encephalopathy, aphasia, dysgraphia, and tremor which should prompt urgent evaluation as they correlate with electrographic seizure activity, cerebral edema, and life-threatening elevated intracranial pressure (ICP). Like the patient in this case, all patients are treated empirically with prophylactic anticonvulsants for the first 30 days after CAR T-cell infusion. Patients who develop signs of neurotoxicity should be promptly evaluated for signs of seizure or cerebral edema. Administration of the anti-IL-6 therapy tocilizumab is used for treating CRS. Prompt initiation of corticosteroids is the treatment of choice for neurotoxicity.
Clinical presentation, grading, and general management of CAR T-cell neurotoxicity
Incidence and risk factors. Along with CRS, the other significant toxicity observed after CAR T-cell therapy is neurotoxicity. The published incidence rates range from 20–64% and occurrence of ICANS may be a negative prognostic factor for overall survival. Although neurotoxicity can occur alone in some patients, this toxicity generally tracks with CRS severity, and both are correlated with enhanced CAR T-cell expansion. Patients at high risk of CAR T-cell–related neurotoxicity are generally the same patients who are at high risk of CRS. Thus, well-established factors associated with elevated risk of neurotoxicity include higher peak CAR T-cell expansion and high pretreatment disease burden. Less well-established factors that may predispose to neurotoxicity include higher infused CAR T-cell dose, high intensity lymphodepletion regimens, preexisting neurologic comorbidities, younger age, and thrombocytopenia, which may be a surrogate for preexisting endothelial activation.
Pathophysiology . The pathophysiologic mechanism underlying CAR T-cell-related neurotoxicity is not fully understood, but passive diffusion of cytokines into the brain, as well as trafficking of T-cells into the CNS, have been postulated. , In humans, it has been shown that endothelial dysfunction and increased blood-brain barrier permeability are present in patients who develop neurotoxicity. In nonhuman primates with CAR T-cell–related neurotoxicity, CAR and non–CAR T-cell infiltration into the CSF and brain, as well as increased levels of proinflammatory cytokines, have been observed.
Laboratory tests and neuroimaging. Correlation between the development of CAR T-cell–related neurotoxicity and laboratory parameters is imperfect, and identification of predictive biomarkers for severe toxicity is needed. One study demonstrated that serum ferritin levels peak with the onset of neurologic symptoms, and higher ferritin levels were associated with higher neurotoxicity grade. As with the patient in the presented case, protein levels in the CSF are often elevated in patients with CAR T-cell–related neurotoxicity, but other CSF studies are typically normal. MRI and CT scan of the brain are usually negative for anatomic pathology. However, cases of reversible T2/FLAIR MRI hyperinstensity and cerebral edema , have been reported.
Signs and symptoms of CAR T-cell–related neurotoxicity. The onset of neurotoxicity from CAR T-cell therapy either occurs:
- 1.
Acutely within the first 5 days after CAR T-cell delivery, often accompanied by fever and other CRS symptoms
- 2.
Delayed neurotoxicity occurring after fever and other CRS symptoms have subsided, more than a week after cell infusion
The clinical presentation of CAR T-cell–related neurotoxicity is similar to that of other toxic encephalopathies. The earliest signs include tremor, dysgraphia, mild expressive aphasia, impaired attention, apraxia, and mild lethargy. Headache sometimes occurs but is not specific for CAR T-cell–related neurotoxicity. Conversely, difficulty with speech, which often begins with mild expressive aphasia and can progress to global aphasia with both expressive and receptive difficulty, is highly specific for this condition. Thus, while certain features of CAR T-cell–related neurotoxicity may overlap with other encephalopathies frequently observed in cancer patients (e.g., hepatic, toxic/metabolic, etc.), an awake patient who is mute and does not respond verbally or physically to an examiner is likely to have neurotoxicity from CAR T-cell therapy. Other symptoms and signs of neurotoxicity from CAR T-cells include confusion, disorientation, agitation, and somnolence. In severe cases, seizures and increased ICP can occur, and these are covered in more detail in subsequent sections of this chapter. Once the first signs of neurotoxicity are observed, progression to more severe symptoms can occur over the course of hours to days.
Grading of CAR T-cell–related neurotoxicity. Neurotoxicity from CAR T-cell therapy was initially graded using Common Terminology Criteria for Adverse Events (CTCAE) criteria. However, as more trials have been conducted and clinical experience with neurotoxicity has increased, more specific grading systems have been published and are increasingly utilized. , First, a multi-institutional group of oncologists leading CAR T-cell trials across the United States published the CARTOX criteria for adults on grading of neurotoxicity ( Table 30.1 ). The CARTOX system grades neurotoxicity by assessing multiple neurologic domains that span the constellation of signs and symptoms associated with neurotoxicity. CARTOX incorporates a 10-point screening tool called the CARTOX-10, which includes the components of the Mini-Mental State Examination (MMSE) that are most specifically affected by the encephalopathy observed in patients with CAR T-cell–related neurotoxicity. The CARTOX grading system also includes other domains, such as level of consciousness, motor symptoms, seizures, and signs of elevated ICP. More recently, Lee et al. published the American Society for Blood and Marrow Transplantation (ASBMT) ICANS grading scheme ( Table 30.1 ). This grading scheme was intended to improve the objectivity, reproducibility, and practicality of assessing neurotoxicity in CAR T-cell–treated patients. The updated encephalopathy grading tool used in the ASBMT ICANS scheme, referred to as the Immune Effector Cell-Associated Encephalopathy (ICE) score, is nearly identical to the original CARTOX-10 scoring system; the main change is the addition of an element for assessing the receptive aphasia that is seen in these patients. In addition to this minor change in the scoring tool, the ASBMT ICANS seizure and ICP grading schema have been simplified ( Table 30.1 ).
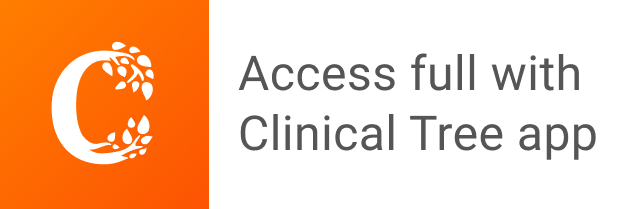