Fig. 19.1
Glucocorticoid mechanisms of action. Mechanisms of glucocorticoid (GC) action. GC crosses the cell membrane and binds to the glucocorticoid receptor (GR) in the cytoplasm. GRs are kept in an inactive state and prevented from moving into the nucleus by heat shock proteins (HSP). Upon binding of a GC to a GR, the HSP dissociates and the GC-GR complex moves to the nucleus via a nuclear pore (NP). The complex then binds to GC responsive elements (GRE) in the 5′ promoter region of DNA. Transcription is then activated. However, if the region contains a negative GRE, transcription is repressed. (Source Salvador et al. [3]. Open Access)
Glucocorticoid Actions on Target Tissues
In order to respond to physiologic and pathologic states, GCs affect nearly every cell of the body and modulate the expression of approximately ten percent of the body’s genes. Actions of particular relevance to this chapter will be divided into effects on general cellular function, inflammation, systemic function, and nervous system function.
General cellular effects of GCs include the promotion of apoptosis and necrosis, which occur through the genomic mechanisms of gene trans-activation and trans-repression by the GR [10, 11]. The apoptotic effect of GCs is cell-type-specific, as well as time- and concentration-dependent [12]. However, the specific mechanisms of GC-induced apoptosis, their target genes, and their respective proteins remain incompletely understood [13–20]. An example of one such protein is NF-kB, whose DNA-independent repression induces apoptosis in many hematologic cell lines [15].
Anti-inflammatory effects of GCs include both the prevention and suppression of the inflammatory response. Specific actions depend on the type and dose of GCs used, the type and location of inflammation, and the type and number of immune cells involved. Anti-inflammatory actions of GCs occur via several mechanisms, including direct and indirect genomic repression. One example of such a mechanism includes the indirect repression of several mediators occurs via inhibition of transcriptional factor NF-kB. Collectively, these mechanisms minimize the proliferation, recruitment, and functional activity of lymphocytes, neutrophils, macrophage, and monocytes by blocking the expression of endothelial and intercellular adhesion molecules, decreasing prostaglandin-mediated cell adhesion, decreasing chemokine binding to leukocytes, and inhibiting plasminogen activator synthesis [21–24]. These mechanisms also minimize the functions of pro-inflammatory mediators such as chemokines, cytokines, adhesion molecules, arachidonic acid metabolites, platelet-activating factor release, and pro-inflammatory enzymes and peptides [25]. The above mechanisms have been preferentially exploited for use in various hematologic and solid malignancies.
Systemic effects of GCs cannot be fully addressed in this chapter; however, those particularly relevant to the treatment and complications of cancer therapy will be addressed in subsequent sections and are listed in Table 19.1. GCs have a multitude of effects on a wide range of organ systems including the vascular, gastrointestinal, hematologic, dermatologic, and metabolic systems. Specific examples of these effects include regulation of blood pressure via the alteration of tissue sensitivity to catecholamine and the aldosterone-like effect on fluid retention, alterations in the number of circulating red blood cells and neutrophils, promotion of protein catabolism, gluconeogenesis, and glycogen synthesis, as well as alteration of lipid and bone metabolism. GCs also regulate protective prostaglandins and cellular integrity in the GI tract and can result in the thinning of epithelial and connective tissue throughout the body, thereby affecting skin integrity.
Table 19.1
Complications of pharmacologic glucocorticoid use
Complications of glucocorticoid use | |
---|---|
Acute | Chronic |
Infectious Bacterial, viral, fungal Neurologic Psychosis, euphoria, anxiety, insomnia, increased appetite Cutaneous Poor wound healing, acneiform eruptions Cardiovascular Fluid retention, arrhythmia Endocrine Hyperglycemia, suppression of adrenal function | Infectious Pneumocystis pneumonia, tuberculosis Neurologic Memory impairment, insomnia, depression, pseudotumor cerebri Cutaneous Striae, purpura, hirsutism, alopecia, non-melanoma skin cancer Cardiovascular Hypertension Endocrine Suppression of adrenal function Musculoskeletal Osteoporosis, myopathy, avascular necrosis, growth retardation Gastrointestinal Peptic ulcer Ocular Cataracts |
Nervous system effects of GCs involve both the central nervous system (CNS) and peripheral nervous system (PNS) levels. Mechanistically, acute exposure to GCs results in neuronal hyper-polarization, which is the result of alterations in intracellular calcium levels, interactions with GC protein-coupled receptors, and induction of MAP kinase cascades. Chronic exposure results in decreased quantity of neurons, induction of apoptotic cell death, impaired regenerative properties, and decreased neuronal survival [26]. Clinically, GC exposure impacts cognition (memory, mood, and personality), sleep patterns, pain perception, body temperature, muscle strength, and vasogenic edema.
Mechanisms of Resistance
Mechanisms of GC resistance remain poorly understood and vary with the genetic background of the patient, steroid type, disease type, and treatment regimen.
One mechanism of GC resistance involves apoptosis , which, amazingly, can produce seemingly dichotomous results. Such dichotomies are epitomized by the paradoxical effects that GCs have across various malignancies, as well as in response to various chemotherapy agents. One such example includes the pro-apoptotic effect of GCs on hematopoietic malignancies (discussed in the Mechanism of Action section), in contrast to the anti-apoptotic effects of GCs on many solid malignancies. For example, Zhang et al. [27] demonstrated dexamethasone and other GCs cause resistance to several cytotoxic chemotherapies and radiation in xenografted prostate cancer cells. A second mechanism of GC resistance involves inhibiting apoptosis-inducing receptors, signaling molecules, caspase enzymes, and mitochondrial membrane stabilizing proteins [28]. For example, dexamethasone has been shown to antagonize both mitochondrial and death receptor apoptotic pathways across various cancer cell lines [28], thus resulting in dexamethasone-induced apoptotic resistance in cell lines of solid malignancies, such as melanoma, neuroblastoma, gliomas, adenocarcinomas (e.g., breast), carcinomas (e.g., cervical), and bone cancers. Interestingly, many of the apoptosis genes repressed by GCs in carcinoma cell lines are the very same genes induced by GCs in hematopoietic malignancy cell lines [28, 29]. These seemingly paradoxical effects have been postulated and include cell-type specific differences in both transcriptional regulation and cell cycle progression, as well as cell-type differences in the development of mutations of GC function [28, 30–34]. A third mechanism of resistance involves perturbations of the GR gene and the GR receptor [32, 35–41]. A fourth mechanism of resistance involves activities essential to epithelial cell survival, including alterations of intercellular communication and extracellular matrix attachment, which have resulted in the chemotherapy resistance of many solid malignancies [42].
A fifth mechanism of resistance involves iatrogenic factors. For example, chronic exposure to GCs reduces both GR expression and GR protein stability [43–46]. In another example, concomitant use of medications that interact with the cytochrome P450 system can reduce the efficacy of GC metabolism and bioavailability [47–49]. In a third example, states of chronic inflammation can reduce the efficacy of GCs through the activation of both GR-dependent and GR-independent signaling pathways. Chronic inflammation can be induced by: (1) cigarette smoking, which induces an oxidative stress that affects both GR nuclear translocation and nuclear cofactors (2) inflammatory conditions such as asthma, which promote immune-mediated cytokines such as IL2 and IL-4, which diminish GR translocation and binding affinity at target cells, (3) viral infections, which reduce both GR nuclear translocation and GC functions, (4) allergen exposures, which affect both GR function and GR-binding affinity, (5) changes in the cellular micro-environment, such as during disease progression, which alter GR translocation and P-glycoprotein-mediated cellular ligand accumulation, (6) oxidative stress, which attenuates histone deacetylase-2 (HDAC2) activity and expression, thereby limiting recruitment of GC to sites of action in the genome by GR, and (7) hypoxia, which induces cellular dysfunction and impairs GR trans-activation [32].
Metabolism of Glucocorticoids
Endogenous
The adrenal cortex is responsible for the production and regulation of corticosteroids. The most peripheral of its three zones, the zona glomerulosa, produces mineralocorticoids, predominantly aldosterone, while the zona fasciculata and reticularis produce GCs and androgens, respectively. These latter two zones respond to pituitary adrenocorticotropic hormone (ACTH) that is secreted into the systemic circulation in response to corticotropin-releasing hormone (CRH) . CRH is released through the pituitary portal circulation by the hypothalamus in response to various physiologic and pathologic stressors.
Corticosteroid synthesis begins with a four-ringed cholesterol substrate, comprising three hexane rings and one pentane ring. The majority of substrate is esterified and stored in the adrenal gland, while a minority is synthesized directly from acetyl-CoA. Upon stimulation by ACTH, an esterase is activated and free cholesterol is released into the mitochondria, where it is converted to pregnenolone by a cytochrome P-450 side chain cleavage enzyme. Pregnenolone is the precursor to all other adrenal hormones, and its fate is carefully controlled by a series of feedback mechanisms. Corticosteroids are differentially synthesized by the sequential action of three hydroxylases at the C11, C17 and C21 positions. The end products include GCs (predominantly cortisol), mineralcorticoids (predominantly aldosterone), and androgens. Cortisol is secreted into the systemic circulation immediately upon production. Only 4–8% circulates in the blood in its active, unbound form. Approximately 92–96% of cortisol circulates in its inactive form, bound mainly to corticosteroid-binding globulin. Adrenal cortisol has a half-life of sixty to ninety minutes in the circulation [50].
Adrenal cortisol secretion is moderated by three main influences. The first influence comes from cortisol’s direct negative feedback inhibition of both CRH and ACTH secretion on the hypothalamus and pituitary, respectively. The second influence comes from the circadian rhythm-related pulsatile release of ACTH that intensifies three to five hours after sleep initiation, peaks at the time of waking, and troughs within an hour or two after sleep initiation. The third influence comes from various physiologic and pathologic stressors, whose release of catecholamine and vasopressin cause the stimulation of hypothalamic-pituitary axis and the release of ACTH. Due to their lipophilicity, endogenous GCs readily cross the plasma membrane and interact with the GC receptor complex, as described above.
Exogenous
Synthetic steroids are manufactured from cholic acid obtained from cattle or from steroid sapogenins (diosgenin) from plants to optimize their bioavailability, plasma half-life, metabolism, and interactions with the GC receptors in target tissues [50]. The time to peak plasma level of most synthetic steroids is between 60 and 90 min, regardless of oral versus parenteral administration and co-administration with food or most other medicines. Most synthetic steroids are transported to the liver where they require metabolism into their active form [51]. Thus, as shown in Table 19.2, the half-lives of various synthetic GCs are significantly affected by the level of overall hepatic and cytochrome p450 enzyme [52–54]. Unlike most endogenous steroids, exogenous steroids tend not to bind to the cortisol binding globulin, but instead circulate attached to either albumin or in its unbound state [55]. This binding difference does not appear to affect the serum half-life of exogenous GCs, which range from about one to four hours. Like endogenous steroids, exogenous GCs readily cross the plasma membrane and interact with steroid receptors in the nucleus. However, the resultant exogenous GC receptor complex produces a two to 11-fold greater effect than its endogenous counterpart. Lastly, as with any agent that acts by affecting nuclear receptors, the disappearance of exogenous GCs does not represent the cessation of effect [56].
Table 19.2
Relative biologic potencies of exogenous (synthetic glucocorticoids)
Equivalency (mg) | Relative potency | Half-life | |||
---|---|---|---|---|---|
Agent | Anti-inflammatory | Mineralocorticoid | Plasma [32] | Biologic (h) | |
Short acting | |||||
Cortisone acetate | 25 | 0.8 | 0.8 | 30 | 8–12 |
Hydrocortisone | 20 | 1 | 1 | 90 | 8–12 |
Intermediate acting | |||||
Prednisone | 5 | 4 | 0.8 | 60 | 12–36 |
Prednisolone | 5 | 4 | 0.8 | 200 | 12–36 |
Methylprednisolone | 4 | 5 | 0.5 | 180 | 12–36 |
Triamcinolone | 4 | 5 | 0 | 300 | 12–36 |
Long acting | |||||
Dexamethasone | 0.75 | 20–30 | 0 | 200 | 36–72 |
Betamethasone | 0.8 | 20–30 | 0 | 300 | 36–72 |
The metabolism of exogenous GCs is clinically relevant in several ways. For example, the modifications that certain exogenous GCs undergo during synthesis result in differential cross-reactivity with the mineralocorticoid receptor, and thus differential complications. Also, requirement of many exogenous GCs to undergo activation in the liver results in the preferential administration of steroids not requiring liver activation, such as prednisolone, to patients with severely compromised liver function. Lastly, the requirement of many exogenous GCs to bind albumin results in higher free GC levels and more side effects in patients with low albumin [57].
Common Therapeutic Uses
General Principles
Despite decades of use in systemic and nervous system conditions, the majority of our clinical knowledge is empiric and not evidence based. Although this has likely limited our optimal use of GCs, the impact of GCs on cancer therapy remains tremendous.
Tumor-Directed Treatment
Exogenous GCs promote death of cancer cells via a variety of mechanisms, including apoptosis. GCs have been historical mainstays in the treatment of systemic solid tumors, including breast, prostate, thymoma, and endocrine-responsive cancers [58]. GCs, with or without concomitant chemotherapy agents, have also been historical mainstays in the treatment of systemic hematologic malignancies , including chronic myelogenous leukemia (CML), multiple myeloma, chronic lymphocytic leukemia (CLL), and virtually all non-Hodgkin’s lymphomas. For instance, GCs provide lifesaving relief to rapidly growing diffuse large B-cell lymphoma affecting the mediastinum [59].
GCs are also instrumental in the control of hematopoetic malignancies affecting the nervous system, presumably due to their previously described lympholytic mechanism, excellent penetration of the blood–brain barrier, and relatively long half-life [1, 60]. For example, dexamethasone, in combination with other chemotherapy agents, has resulted in improved CNS control of acute lymphoblastic leukemia, which has a high predilection for the CNS, and primary CNS lymphoma (PCNSL) [61, 62]. Rapid initial clinical response is a hallmark of GCs in hematologic malignancies affecting the nervous system, secondary to their ability to induce apoptosis in a p38 mitogen-activated protein kinase (MAPK)-dependent manner in B and T cells [63]. However, the effects of steroids are too transient and inefficient not to be coupled to radiotherapy and/or chemotherapy. GCs also indirectly provide anti-neoplastic effects to tumors being treated by allogeneic hematopoetic stem cell transplants by helping to prevent transplant rejection as well as prevent graft-versus-host disease [64].
Historically, there has been no clinical evidence that steroids inhibit the growth of gliomas or metastases in human patients. However, data from preclinical studies suggest that the invasiveness and proliferation of some glioma cells may be reduced by exposure to dexamethasone [65, 66]. GCs should be avoided in the initial management of patients suspected of having a hematologic malignancy of the nervous system, such as PCNSL, or infection, whenever clinically safe, as their lympholytic effects can obscure the histopathologic diagnosis. Fortunately, techniques to improve analysis yield by spinning down a CSF cytospin into a cell block, as well as the availability of other tests highly relevant to PCNSL, such as EBV, clonal heavy chain gene rearrangement, and pathologic expression of BCL2 and BCL-6 family proteins, are overcoming this historic challenge [67].
The emerging field of cancer immunotherapy is elucidating the profound complexities of the body’s immune system, including the ability to harness it to fight nervous system and systemic malignancies. In brief, immunotherapy is an attempt to correct a failed or inadequate immune system, whether caused by innate, acquired, or iatrogenic immunosuppression, or by a tumor or a tumor-associated virus. Historical agents include IL-2 and interferon, and newer agents include effectors of checkpoints on T cells: PD-1, PD1-Ligand, CTLA-4, etc. Thus, understanding the interplay of GC immunomodulatory capabilities is paramount. Despite an incomplete translational understanding, several clinical trends have manifested. First, there exists the recommendation for using the “least clinically needed” GC dose during treatment with immunotherapy. Second is the use of GCs to suppress the often profound inflammatory sequela of immunotherapy. This can not only be lifesaving, but also allow for eventual resumption of treatment. A severe immune reaction necessitating prolonged GC use may require consideration of infliximab. Information about the immune-related toxicities of immunotherapy agents, which are measured by NCI’s Common Toxicity Criteria [68] can be found within product inserts, FDA product safety releases, and Risk Evaluation and Management Strategies. Third, in available studies and practical experience, the judicious use of GCs with immunotherapy has not shown deleterious efficacy or overall survival. Lastly, opportunistic infection, such as Aspergillus pneumonia, Fournier’s gangrene, Pneumocystis jiroveci pneumonia, cytomegalovirus colitis, and mucor mycosis, is another comorbidity of both GC use and immunotherapy that clinicians must identify and manage aggressively [69].
GCs should always be maintained at the lowest clinically needed dose, not only to minimize side effects, but also because numerous translational and clinical studies across tumor types suggest that GCs may result in chemotherapy resistance, via a variety of mechanisms [70].
Symptom-Directed Treatment
GCs serve a major role in cancer therapy by increasing the safety and tolerance of many chemotherapies, including cytotoxic drugs, monoclonal antibodies, immunotherapy, and other agents.
Anti-Emetic
One of the most important uses of GCs is the prevention or reduction in anticipatory nausea, a complex psychological–physiological phenomenon where patients experience nausea and vomiting at the mere memory trigger of the chemotherapy experience [71]. Mechanisms for these effects are not fully elucidated; however, reduced release of 5-HT3 from blood mononuclear cells upon administration of steroids as well as direct inhibitory effect on 5-HT3 receptor have been suggested as important factors [72, 73]. Furthermore, animal study data suggest a direct effect of corticosteroids in the medulla oblongata [74]. Methylprednisolone and dexamethasone have been shown to directly antagonize the serotonin receptors and GR associated decreased prostaglandin synthesis at target tissue [75].
Improve Tolerance of Treatments
GCs are used in numerous chemotherapy preparatory regimens ameliorate the symptoms associated with hypersensitivity. For example, GCs prevent various hypersensitivity reactions known to occur with certain chemotherapies and monoclonal antibodies [76]. GCs are used to reduce the hematologic toxicity associated with various chemotherapies or even the cancer itself. For example, GCs have been shown to reduce hematologic toxicity in advanced breast cancer patients receiving GCs concurrently with chemotherapy and reduce the severity of autoimmune conditions related to cancer or its treatments, including autoimmune hemolytic anemia or thrombocytopenia [58]. GCs are used to treat lethargy (fatigue), weakness, anorexia and can even induce a short-term euphoria [1]. GCs are used in the treatment of hypercalcemia associated with cancer when bisphosphonates are either contraindicated or ineffective [77]. GCs are also used to alleviate pain associated with bone metastases and their treatments, likely through the reduction in peri-neural edema and the inhibition of prostaglandin synthesis [78].
Anti-Inflammatory
GCs are frequently used to treat dyspnea, pleural effusion, ascites, pleuritis, lymphangitic carcinomatosis, and hemoptysis. This anti-inflammatory effect is caused, in part, by a reduction in the levels of eosinophils, monocytes and lymphocytes (despite a paradoxical increase in neutrophils), and an impairment of their function [79]. Similarly, GCs prevent or minimize a variety of cutaneous syndromes, including rashes, resulting from chemotherapy [80]. Lastly, GCs have been shown to ameliorate a symptomatic immune reaction in patients receiving immunotherapy for CNS and systemic malignancies, and to date, without documented diminution in their effects on tumor control and survival [69].
Anti-Edema
Since their first reported use for post-operative cerebral vasogenic edema in 1952, GCs have been mainstays in the treatment of vasogenic edema from primary and metastatic tumors throughout the nervous system [81–83]. Vasogenic edema is caused by a disrupted blood-brain barrier (BBB), resulting from breakdown of inter-endothelial tight junctions, increased endothelial pinocytosis, and increased endothelial fenestrations. Though the mechanism of BBB disruption remains unclear, VEGF release and tumor under expression of tight junction proteins likely play a role [84–86]. Steroids decrease the permeability of the blood brain barrier and limit the extravasation of fluid. This effect is mediated in part by the induction of occludin, transendothelial electrical resistance (TER) and zonula occludens (ZO)-1 expression. An increase in claudin-5 promoter activity and mRNA expression, as well as a decrease in expression of vascular endothelial-cadherin, intercellular adhesion molecule-1 (ICAM-1) and vascular cell adhesion molecule-1 (VCAM-1) , are also involved [87, 88].
The GCdexamethasone has been shown to ameliorate symptoms of vasogenic edema in over 70% of both primary and metastatic CNS cancer patients, including headache, somnolence, confusion, and to a lesser extent, seizure and focal neurologic deficits [2]. High-dose dexamathasone can also reverse life-threatening effects of vasogenic edema such as cerebral herniation or neoplastic epidural spinal cord compression (ESCC) [89, 90].
Given the desire to minimize GC side effects, dexamethasone “alternatives” continue to be explored, including corticotrophin-releasing factor, carbonic anhydrase inhibitors (e.g., acetazolamide), diuretics (e.g., furosemide, mannitol), and VEGFR-modulators (e.g., bevacizumab), and many others—discussed later in this chapter. To date, none have completely replaced GCs.
Common Therapeutic Regimens
General Principles
The determinants of GC dosing include pharmacokinetics of the different drug preparations, effects of underlying disorders on drug kinetics, and interactions of GCs with concurrently administered non-GC drugs. In addition, GCs must be individualized to specific target tissues, conditions and patient’s organ function and comorbidities.
In addition, GCs cannot be titrated optimally without knowledge of how to monitor drug activity and how they impact each organ. For example, GCs significantly impact the ability for contrast dye to cross the blood-brain barrier in neuro-imaging studies. As a result, the dose and duration of GCs must be carefully taken into account when scans are interpreted [91, 92] (Fig. 19.2a–d). Furthermore, GCs cannot be titrated optimally without knowledge regarding how to safely withdraw steroids (discussed below) [93, 94].


Fig. 19.2
Serial MRIs of metastatic melanoma patient presenting with acute neurologic decline and showing reduction in edema and enhancement after 16 mg oral glucocorticoid administration for several days. Axial T2-weighted pre-steroids (a) and post-steroids (b). Axial T1-weighted pre-steroids (c) (attention to the blunting of left rostral ventricle and the effacement of the gyri) and post-steroids (d) (attention to the resumption of the anatomy of the ventricle and the gyri spaces)
Dexamethasone is the mainstay in primary and metastatic CNS cancers, given its minimal mineralocorticoid effects (e.g., less fluid retention, electrolyte imbalances) and long half-life, which allows for administration of a single daily dose [62]. Because of the unavoidable side effects of steroids, the “least clinically needed” dose and duration should always be attempted. Discontinuation must be gradual to prevent withdrawal symptoms.
Anti-Edema
Neurologic symptoms from cerebral vasogenic edema typically improve within 48 h and often within 12–24 h of steroid administration [95]. This is notably sooner than the improvements on MRI and CT imaging, which can take up to 48–72 h. Insufficient benefit either signifies an alternative mechanism of dysfunction or the need for additional therapies, including urgent surgical intervention, hyperosmotic mannitol [81], as well as other “dexamethasone alternatives” listed in the above section.
For patients with highly symptomatic cerebral edema, an initial dose of 16 mg/day is typically used. This dose was empirically established in the 1960s and continues to be recommended [96–99], despite a lack of prospective clinical trial evidence to support it use. In the setting of severe signs and symptoms, a loading dose of 10–20 mg of dexamethasone is often administered intravenously [100]. The bioavailability of IV and oral formulations are equivalent but GI absorption is usually 30–60 min. Maintenance doses of dexamethasone are commonly 4–24 mg daily. Importantly, given its long biologic half-life of 36–54 h, dosing more than once or twice daily is unnecessary, with administration times preferably in the morning to, the latest, early afternoon. This is particularly important for compliance and quality of life of patients after hospital discharge. For additional management principles and applications of GCs in CNS cancers, readers are directed to comprehensive reviews by Kostaras et al. [93], Ryken et al. [60], and Roth et al. [62].
For patients with highly symptomatic spinal cord edema, such as from a tumor, immediate commencement of dexamethasone at 50–100 mg is recommended, followed by 16 mg daily [89, 90]. The onset and degree of symptomatic improvement, usually beginning within several hours, helps to determine the urgency of surgical intervention or other treatments.
Ameliorating Symptoms
GCs use to increase the safety and tolerance of many chemotherapy agents, which is highly individualized. For example, pre-medication for chemotherapy usually involves a one-time dose of 10 mg IV dexamethasone. The steroid is administered at least thirty minutes prior to the chemotherapy and is used to prevent acute transfusion reactions, anaphylaxis, or severe nausea. Patients on chemotherapy agents known to cause a more protracted cutaneous toxicity or nausea are often prescribed oral dexamethasone between 1 and 4 mg twice daily for three to five days prior to and after chemotherapy, often in combination with other supportive medications [75]. For the treatment of immunotherapy toxicity, dexamethasone is used at a dose ranging from 4 to 24 mg/day, and sometimes exchanged for once or biweekly infliximab [69]. Similar dosing can be used to treat other inflammatory conditions, including pleuritis, ascites, hemolytic anemia associated with malignancy, though prednisone is often favored over dexamethasone.
Tumor-Directed Treatment
GCs are used for their lympholytic properties in hematologic malignancies that affect the nervous system, including PCNSL and systemic lymphomas and leukemias with CNS involvement. Importantly, if the initial diagnosis of suspected lymphoma or leukemia is being investigated, dexamethasone should be withheld unless signs and symptoms are significant. This recommendation results from the disappearance of classic imaging or pathologic features that often occur after rapid and significant lympholysis. Although advances in imaging and pathology are minimizing this necessity, the general recommendation remains. Once the tumor has been diagnosed, starting doses usually range from 4 to 16 mg of dexamethasone a day. Similarly, GCs remain important components of regimens for various systemic and hematopoetic malignancies, both for their anti-neoplastic as well as symptom–amelioration roles. Across malignancies, the choice of GC, route of administration, dose and duration are highly individualized and, therefore, must be reviewed prior to prescription.
Tapering Regimens
Safe and optimal tapering involves knowledge of the dose, duration, drug activity, and status of the condition for which the GCs was used. The goal of tapering is to prevent an exacerbation of the underlying condition as well as prevent symptomatic adrenal insufficiency, a.k.a. steroid withdrawal symptoms, characterized by nausea, headache, myalgias, anorexia, and diverse nervous system symptoms [101]. Monitoring of tapering regimens predominantly involve close evaluations of signs and symptoms of withdrawl, but may include laboratory and imaging tests.
An attempt at tapering should be made in all patients and anticipation of the re-emergence of symptoms should not deter attempts [102]. Tapering of dexamethasone usually occurs over several weeks. For example, patients with brain tumors who have undergone gross or near total resections can usually be tapered down to off within a week or two after surgery and rarely need dexamethasone during radiotherapy. In contrast, brain tumor patients who have minimal or no surgical debulking usually require dexamethasone throughout radiotherapy. Spine tumor patients are generally recommended to maintain dexamethasone throughout radiotherapy and can usually be tapered down to off within two to three weeks depending on neurologic improvement. All patients should be observed for symptoms of adrenal insufficiency if dexamethasone is discontinued abruptly or used for a prolonged time, and advice from an endocrinologist should be sought if needed.
Despite the paucity of prospective, randomized trials, various tapering protocols are used, as illustrated in Table 19.3. For dexamethasone, the duration of one month at moderate dose is generally accepted as the threshold to recommend a tapering regimen. The attempted rate of tapering should be fast in the first 10 days of corticosteroid therapy (e.g., every 1–3 days) but slower after this (e.g., every 4–7 days, and even slower once physiological doses are reached). One commonly used strategy is to reduce the total daily dose by 25% each week; however, a more gradual discontinuance over 8–12 weeks may be needed for patients who have received dexamethasone for long periods of time.
Table 19.3
Suggested glucocorticoid tapering strategies
Duration | <1 montha | 1 month—1 yearc | >1 yearc |
---|---|---|---|
Tapering strategy | Can stop immediatelya | Reduce dose by 25% each week over four weeksa,c | Taper first 75% of dose over a month, and the residual over another four weeksa,c |
If steroids have been used for more than a few weeks, then assessing for occult hypocorticolism is prudent. Baseline fasting morning (basal) cortisol levels can be tested before steroids have stopped. Patients with low cortisol levels benefit from replacement with hydrocortisone, with the first dose, (usually ~20 mg) given in the morning, and the second dose, (usually ~10 mg) at noon, to mimic the physiological secretion of the hormone [93, 103]. See additional information in the section below, entitled Management of Adverse Events Associated with Dexamethasone Therapy.
Common Non-Neurologic Clinical Toxicities
In general, GC side effects are common, correlated to dose and duration. For example, in a chart review of 88 patients with brain metastases, Sturdza et al. [97] reported that 91% of the patients receiving 16 mg or more daily and 65% of the patients receiving less than 16 mg daily experienced at least one side effect (p = 0.006). Hypoalbuminemia exacerbates GC side effects because such a state results in an increased percentage of unbound steroid [102].
Management of Adverse Events Associated with Dexamethasone Therapy
Drug–Drug Interactions
Exogenous GCs co-administered with other drugs (including topical and mucosal preparations) can produce drug–drug interactions as a results of disrupted globulin binding or induction/inhibition the hepatic p450 system enzymes. For example, enzyme-inducing medications such as rifampin, phenytoin, carbamazepine, and barbituates can stimulate the metabolism of GCs and thus require an increased dose to achieve the desired effect [101, 104]. Alternatively, ketaconazole and many hormone-containing oral contraceptives can cause a 50% higher dose of unbound GCs concentration [105].
Glucocorticoid-Induced Adrenal Insufficiency
Prolonged exogenous GC use leads to atrophy of the zona fasciculata layer of the adrenal gland. Abrupt cessation or insufficient tapering can result in either symptomatic acute or insidious adrenal insufficiency (AI), often termed steroid withdrawal syndrome. Suppression of hypothalamic-pituitary-adrenal function by chronic administration of high doses of GCs is the most common cause. The presentation, diagnosis, and management of GC-induced AI are briefly summarized below.
The adrenal gland secretes ~20 mg of cortisol daily, and thus GC administration at doses greater than what is equivalent to ~5 mg of prednisone daily could cause suppression of the hypothalamic-pituitary-adrenal axis, a.k.a. secondary adrenal insufficiency (AI). Importantly, this can occur with any route of GC administration, including topical and inhaled. Because the adrenal gland recovers quickly from suppression, even after large doses, the use of GCs for less than a week is unlikely to cause AI with abrupt withdrawal. In contrast, patients receiving doses of ~20 mg of prednisone daily (or other steroid equivalent), those receiving doses in the evenings for more than a few weeks, and those with a cushingoid appearance, are recommended to undergo a tapering regimen to prevent symptomatic steroid withdrawal syndrome [106].
When evaluating a patient for suspected AI, it is important to distinguish primary AI, such as caused by a pituitary tumor, from secondary AI, such as caused by insufficient GC tapering, because the evaluation and treatment can differ. In primary AI, cortisol secretion is deficient despite the ability to secrete ACTH. In secondary AI, deficient ACTH secretion results in atrophy of the zona fasciculata and zona reticularis of the adrenal gland and impaired cortisol production and secretion. Another important difference is that secondary AI maintains nearly normal mineralocorticoid secretion because this function depends mostly on the renin-angiotensin system rather than on ACTH. Collectively, these differences form the basis of the laboratory confirmation of AI, and ultimately, their management.
The most common clinical features of secondary AI caused by GCs are listed in Table 19.1 [107], and include nausea, vomiting, abdominal pain, fever, confusion or coma, myalgia, arthralgia, and psychiatric symptoms, chronic fatigue worsened by exertion and improved with rest, generalized weakness, anorexia, weight loss, and in the case of adrenal crisis- hemodynamic shock. Patients with primary AI will also have symptoms of mineralocorticoid deficiency, including abnormal electrolytes, hemodynamics, hyperpigmentation (because ACTH secretion is increased), and in women, androgen deficiency.
Distinguishing between primary and secondary AI requires a thorough H&P and complementary laboratory tests. A comprehensive review of laboratory tests for the evaluation of AI is beyond the scope of this chapter, but given the nuances of these laboratory tests and the variable presentations of patients, the early involvement of endocrinology is often very helpful. Notably, laboratory tests prior to urgent treatment may not always be practical, and work-up for possible causes of AI should be considered, including evaluation for infection, bleeding, or metastatic disease, which may require their own urgent empiric treatment.
Patients in adrenal crisis or those with AI and undergoing physiologic stress, such as trauma or surgery, frequently require treatment with hydrocortisone at 10–15 mg/m2 (~100 mg) IV of body surface area daily (a.k.a. stress dose steroids). Equivalent doses of IV dexamethasone are favored if cortisol assays for adrenal function testing are being considered. Patients may also require aggressive hemodynamic support, intensive care monitoring, and evaluation and treatment for possible causes of AI [108]. A response is typically seen within 4–6 h of initiating treatment. Patients not presenting in crisis may be empirically treated with dexamethasone, usually 4–16 mg PO daily [109].
Infection
GC exposure can increase the risk of infection by Pneumocystis jirovecii pneumonia (PJP) (formally known as PCP), Listeria, Legionella, cryptococcus, cytomegalovirus, and many other organisms [110–113]. Furthermore, GCs can mask the features of acute infection, since symptoms such as erythema, swelling, and pain are dependent on the presence of functioning leukocytes. Lastly, they can falsely simulate true infection by the neutrophilia caused by de-margination. In this situation, the presence of more than 6% bandemia substantiates true infection [114].
There are no prospective trials or guidelines for the prevent of infection with GC use in malignancy; however, common wisdom recommends minimizing the burden of malignancy contributing to immunosuppression, using the least dose/duration of GCs clinically necessary, and having a low threshold for investigation of common and opportunistic organisms. Tests of exposure, such as for tuberculosis, and vaccinations are not routinely recommended as little is known about the patient’s ability to mount a response [115]. The most evidence-based recommendation for patient with brain tumors receiving GCs for more than six weeks is the use of PJP prophylaxis with trimethoprim/sulfamethoxazole, dapsone, or aerosolized pentamidine, as illustrated in Table 19.4 [116, 117].
Table 19.4
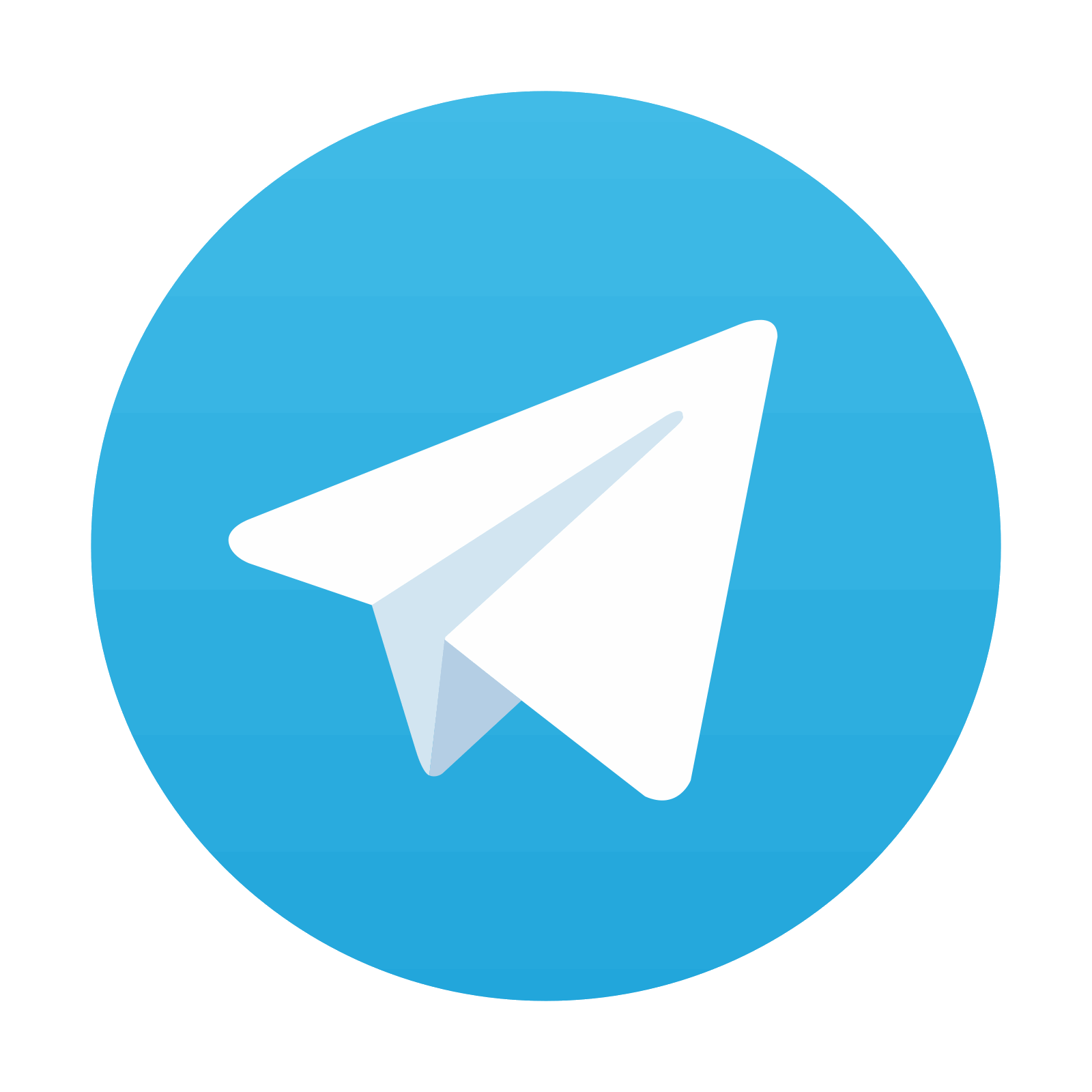
Suggested prophylactic strategies for patients on long-term glucocorticoids
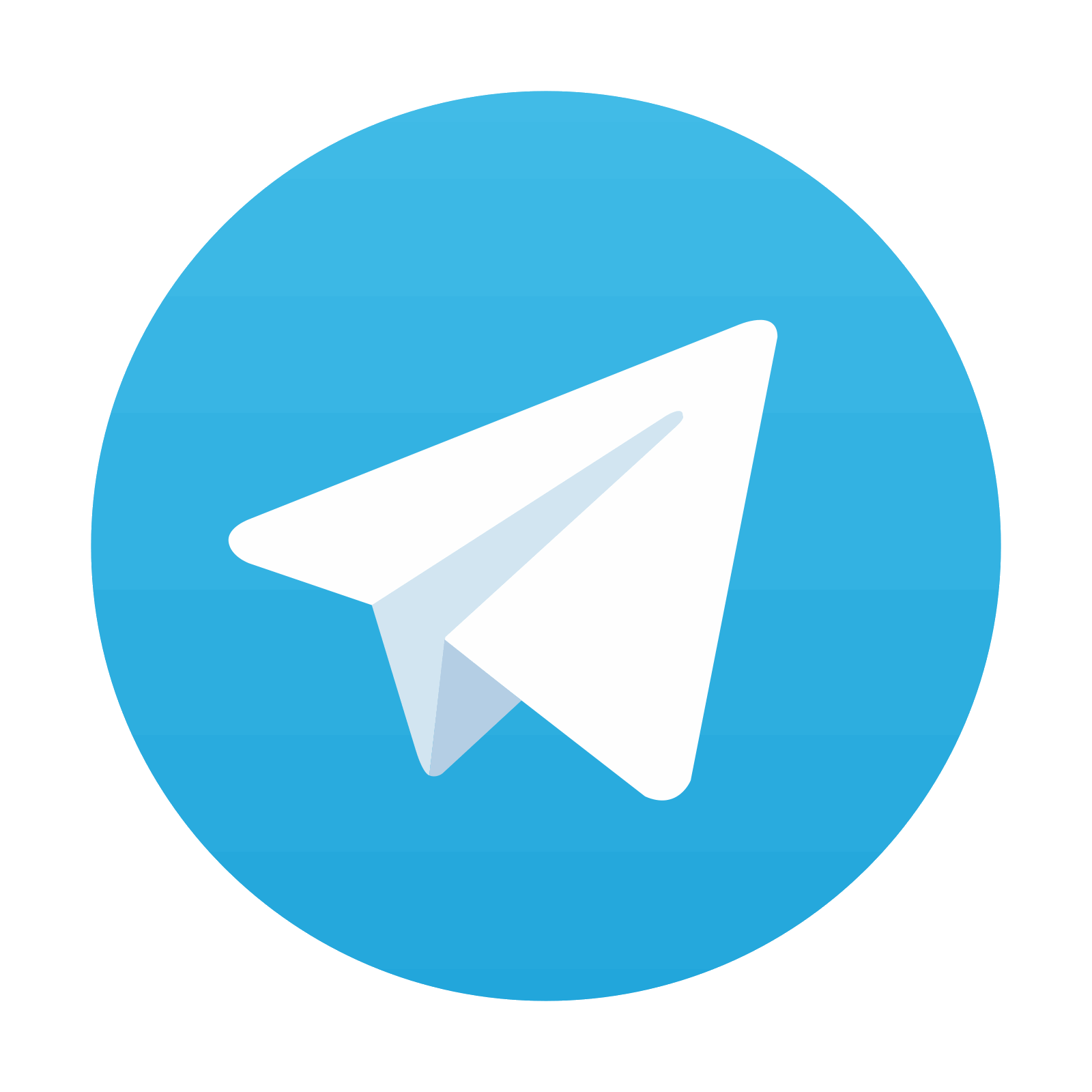
Stay updated, free articles. Join our Telegram channel
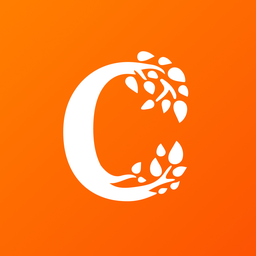
Full access? Get Clinical Tree
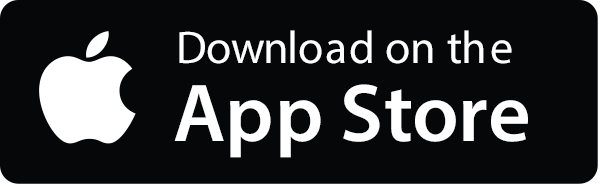
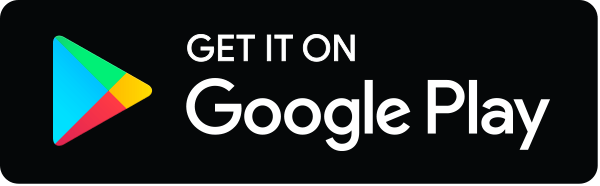
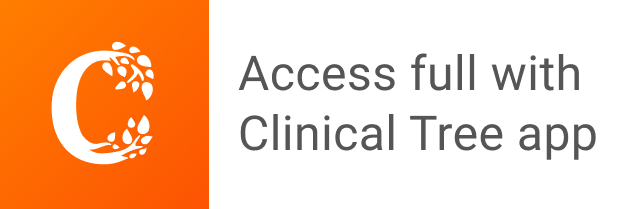