Site
Acute complication (<4 weeks after RT)
Early-delayed complications (1–6 month after RT)
Late-delayed complications (>6 month after RT)
Brain
• Acute encephalopathy
• Somnolence syndrome
• Worsening of pre-existing symptoms
• Transient cognitive impairments
• Subacute rhombencephalitis
• Focal brain radionecrosis
• Cognitive impairment and leukoencephalopathy
• Secondary brain tumors
Spinal cord
• Lhermitte’s sign
• Focal spinal radionecrosis
• Progressive myelopathy
• Spinal hemorrhage
Cranial nerves
• Transient hearing loss
• Anosmia
• Ageusia
• Hearing loss
• Visual loss
• Lower cranial nerves palsies
Peripheral nerves
• Paresthesiae
• Brachial or lumbosacral plexopathy (transient)
• Brachial or lumbosacral plexopathy
• Malignant nerve sheath tumors
• Lower motor neuron syndrome
Sequelae of Radiation Therapy to the Brain
Acute Encephalopathy
Acute encephalopathy usually appears within 2 weeks after the beginning of cranial RT, often a few hours after delivery of the first fraction. The patient presents with nausea and vomiting, drowsiness, headache, dysarthria, and a worsening of pre-existing neurological deficits, sometimes associated with fever. T2 hyperintensities on MRI may be seen according to a recent case report [4]. The clinical course is usually favorable, but herniation and death have been reported in patients with large tumors who have already presented with intracranial hypertension (e.g., with multiple metastases, posterior fossa, or intraventricular tumors). Large doses per fraction (usually >3 Gy/fraction) are the main risk factor: Young et al. [5] reported acute radiation damage in 50% of patients with brain metastases treated with 15 Gy in two fractions to the whole brain, and Hindo et al. [6] reported four deaths within 48 h of a 10 Gy RT given in one fraction. As these large doses are no longer in use, this syndrome is rarely seen. However, a minor form of this condition occurs in many patients, consisting of nausea and moderate headache occurring within hours following cranial irradiation. The pathophysiology of acute complications supposedly results from radiation-induced blood–brain barrier (BBB) disruption, resulting in a rise in intracranial pressure [7]. Steroids may help in preventing or limiting the consequences of acute encephalopathy, especially in patients with large primary or secondary brain tumors or with considerable edema particularly at risk of herniation. In such patients, daily doses of steroids of at least 16 mg dexamethasone should be prescribed 48–72 h before the first fraction; a limited dose per fraction (2 Gy or less per fraction) is also recommended in this situation [8, 9]. An encouraging double-blind randomized placebo-controlled trial has demonstrated in 44 patients that Boswellia serrata derived from Indian frankincense may protect against radiotherapy-induced acute edema [10]. To prevent this complication, surgical debulking should ideally be carried out before starting RT treatment.
Early-Delayed Complications of RT
Several early-delayed clinical patterns have been described (Table 14.1). They occur 2 weeks to 6 months after RT. The pathophysiology is thought to a transient demyelinating process triggered by BBB disruption and/or selective oligodendrocyte dysfunction.
Somnolence Syndrome
This condition was first described in the late 1920s in children receiving low-dose RT for scalp ringworm. Several studies reported cases of children who developed somnolence syndrome 5–8 weeks after prophylactic cranial RT for leukemia [11, 12]; other reports have shown that it also occurs in adults. The incidence of somnolence syndrome varies greatly (with figures ranging from 8 [12] to 84% [13]); this difference is related to various factors including tumor type, radiation dose, fractionation, and diagnostic criteria [14]. Prominent symptoms include drowsiness, somnolence, nausea, and anorexia. Headache and/or fever may also be reported. Friends or family often notice irritability, attentional deficits, and short-term memory impairment. The clinical severity of this complication is variable, with extremes ranging from minimal disorders to sleeping >20 h/day [12]. MRI studies are not contributory. Electroencephalographic abnormalities include nonspecific diffuse slow waves [14]. Most studies report a monophasic course of symptoms, usually with a favorable outcome within a few weeks. However, a prospective study of 19 adults treated for primary brain tumors with cranial RT (45–55 Gy) reported a biphasic pattern of symptoms with two critical periods (from the 11th to the 21st day and from the 31st to the 35th day) following RT [15]. Furthermore, in this study, an accelerated fractionation led to significantly more severe drowsiness and fatigue than a conventional scheme. Some authors advocate the use of steroids during and after radiotherapy as prophylactic or symptomatic treatment [16]. A prospective double-blind randomized trial in leukemic children found that a dose of 4 mg/m2 of dexamethasone during cranial radiotherapy reduced the incidence of somnolence syndrome (17.6% vs. 64.3%) as compared to a dose of 2 mg/m2 [17].
Worsening of Pre-existing Symptoms, or Tumor Pseudoprogression
In patients under treatment for malignant brain tumors, a worsening of pre-existing neurological focal deficits leads to concerns of tumor progression, especially when observed in association with features of the somnolence syndrome and with transitory cognitive impairment. This complication arises within 6 weeks to 3 months after RT, and is clinically impossible to differentiate from progressive disease. Neuroimaging may be normal or show edema and increased contrast enhancement within the tumor bed, a situation that does not allow this syndrome to be differentiated from tumor recurrence and explains why inclusion of patients in experimental regimens for “recurrence” is not indicated during this period [18]; it is worthwhile noting that this radiological pattern can also be associated with no clinical worsening. Pseudoprogression rates can reach 20% after concomitant temozolomide and radiotherapy regimen used to treat glioblastoma and may be associated with better long-term tumor control tumor [19]. Improvement usually follows within a few weeks or months and a follow-up MR scan will commonly show spontaneous regression within 4–8 weeks (Fig. 14.1a–h). As in the somnolence syndrome, the treatment relies upon supportive care with steroids. Improvements in imaging techniques such as magnetic resonance spectroscopy (MRS) and perfusion may help in distinguishing between pseudoprogression and true progression with signs such as subependymal spread of the enhancing lesion [20] but is limited by the fact that residual tumor is often still present within the irradiated area [21].


Fig. 14.1
a–h Tumor “pseudoprogression”. A 59-year-old patient treated by surgery followed by radiochemotherapy for a left temporo-occipital glioblastoma. 2 months after the end of radiochemotherapy, MRI suggested tumor progression based on the increased tumor volume. The tumor volume decreased 3 months later. a Postsurgery axial T1 gadolinium. b Postsurgery axial T2 FLAIR. c 2 months post-radiochemotherapy axial T1 gadolinium. d 2 months post-radiochemotherapy axial T2 FLAIR. e 2 months post-radiochemotherapy perfusion-weighted curves showed an absence of neoangiogenesis. f 2 months post-radiochemotherapy axial perfusion-weighted image. g 5 months post-radiochemotherapy axial T1 gadolinium. h 5 months post-radiochemotherapy axial T2 FLAIR
Transient Cognitive Decline
A transient cognitive decline can be observed within the first 6 months after cranial RT, mainly affecting attention and recent memory, and may sometimes be associated with a somnolence syndrome. Armstrong et al. [22] prospectively followed 5 patients treated for primary brain tumors: memory impairment was conspicuous in all patients 1.5 months after focal cranial RT (43–63 Gy), but complete regression was observed after 2.5–10.5 months.
In another prospective study by Vigliani et al. [23] comparing 17 patients treated with focal cranial RT (54 Gy) for good prognosis gliomas and 14 matched control patients who did not undergo RT, 36% of the patients had a significant early-delayed impairment of their reaction test, with a return to normal baseline performance 12 months after RT. This test result was correlated with their occupational status: 69% of the patients could not work at 6 months, whereas 73% had continued or resumed their job at 1 year.
A recent study [24] investigated the cognitive status and radiological abnormalities during the first months after radiochemotherapy in 39 high-grade glioma patients. Seventy per cent of patients experienced cognitive stability or improvement whereas 30% declined. However, 80% of the decliners showed tumor progression during the following 4 months. The authors could not demonstrate a clear association between cognitive impairments and post-treatment radiographic changes in this study. Hahn et al. [25] showed a dose-dependent correlation between metabolism decrease in brain areas treated with >40 Gy and performances in problem solving and cognitive flexibility during the first 6 months after radiotherapy for CNS tumors.
However, transient cognitive decline is not always observed [26–28] and seems to vary according to patient and mental function involved. In our experience, informing patients about the possible difficulty in returning to a normal life (particularly work) at least during the first 6 months following radiotherapy is useful. Brain irradiation patterns limiting the hippocampal dose may limit subacute cognitive decline in brain and head and neck tumor patients [29, 30]. Although severe and, in some cases, persistent early-delayed symptoms have been occasionally reported [31], it is of note that transient cognitive impairment does not appear to be a clear-cut predictive factor for subsequent development of long-term cognitive disorders.
Subacute Rhombencephalitis
Distinct from brainstem radionecrosis, which occurs later, early-delayed subacute rhombencephalitis may be observed 1–3 months after RT using portals involving the brainstem, as for ocular, pituitary, or head and neck tumors. The clinical picture includes ataxia, dysarthria, diplopia, and/or nystagmus as well as auditory loss. In some cases, the cerebrospinal fluid analysis shows inflammatory changes. MRI may demonstrate white matter abnormalities appearing as grossly round or more extensive T1-weighted hypointensities and T2-weighted hyperintensities affecting the brain stem and cerebellar peduncles; the lesions may enhance after gadolinium injection [32, 33]. The condition usually improves progressively over a few weeks to months, either spontaneously or with steroids, but coma and death have been reported in rare cases [34, 35].
Late-Delayed Complications of RT
Two principal delayed complications may follow RT, usually after more than 6 months: focal radionecrosis and mild-to-severe cognitive impairment associated with leucoencephalopathy. These adverse effects may occasionally be delayed many years.
Focal Brain Radionecrosis
Focal radionecrosis constitutes a challenging complication of radiation therapy because it appears as a bulky contrast-enhancing lesion often mimicking tumor recurrence and its functional consequences can be devastating. This complication may occur not only in patients who received focal RT for a primary or metastatic brain tumor but also in patients without brain lesions with a history of RT for extraparenchymal lesions, in whom normal brain was included in the radiation field (e.g., head and neck or pituitary tumors, meningiomas, skull osteosarcomas). A classic example is the bilateral medial temporal lobe necrosis that results from RT for pituitary or nasopharyngeal tumors (Fig. 14.2a–d). This once frequent complication of conventional RT has become rare over the last 20 years, as a consequence of the generalized use of safer radiation protocols. It has been shown that the upper limits of a “safe dose” were defined by a total dose of 55–60 Gy administered to a focal field with fractions of 1.8–2 Gy per day [36]. The chief risk factors are age at radiation and concurrent chemotherapy with a 5-year toxicity ranging from 27 to 37% in patients with nasopharyngeal carcinomas [37]. The focal delivery of a single large radiation fraction during “radiosurgery ” may also lead to focal necrosis of the brain adjacent to the irradiated lesion [38]. In arteriovenous malformations (AVM) , the incidence of brain necrosis ranges from <5 to 20% of cases, with location and volume found to be the main risk factors [39–41] with an individual and unpredictable sensitivity to ionizing radiation [42]. After standard RT, radiation necrosis generally occurs within 1–2 years [43], but it has been observed after several decades. As described above, shorter latencies (as short as 3 months) have also been reported, especially in patients treated with interstitial brachytherapy [44] or radiosurgery.


Fig. 14.2
a–d Bi-temporal radionecrosis. a 69-year-old patient treated for a clival chordoma with radiotherapy (20 fractions of 1.8 Gy with tomotherapy and 21 fractions of 1.8 Gy with proton therapy). 3 years later, the patient developed memory impairment. a Axial T1 gadolinium shows large heterogeneous contrast-enhanced lesion in each temporal lobe. b Axial T2 FLAIR is suggestive of perilesionnal edema. c Axial T2* shows some microbleeds. d Axial perfusion-weighted image indicates no neo-angiogenesis
Histopathologically , focal brain radionecrosis is characterized by prominent vascular damages including vascular fibrosis/hyalinization with luminal stenosis, thrombosis, hemorrhage, fibrinous exudates, telangiectasias, and fibrinoid vascular necrosis (Fig. 14.3a–d) [45]. These features are associated with edema of brain parenchyma due to disruptions in the blood–brain barrier and parenchymal necrosis. Other common histopathological findings are calcifications, histiocytic infiltrates, and hyalinized and dilated blood vessels with enlargement of parenchymal Virchow–Robin spaces (Fig. 14.4a–d) [45]. The brain parenchyma often contains dystrophic neurons and reactive astrocytes that may be very atypical with pleomorphism and bizarre nuclei [45]. Those changes may raise the differential diagnosis of radiation-induced glioma. Nevertheless, those findings are not associated with high mitotic index or high MIB-1 labeling index as a tumor would have.



Fig. 14.3
a–d Focal brain radionecrosis. Histopathological findings of focal brain radionecrosis. a Hyperplasia of thickened and hyalinized vessels (HES, 60× magnification). b Telangiectatic proliferation of vessels with hemorrhage and some inflammatory cells (HES, 70× magnification). c Hyaline necrosis with ghostly vessels (HES, 70× magnification). d Pallor of the white matter due to loss of myelin (HES, 40× magnification)

Fig. 14.4
a–d Focal brain radionecrosis. Histopathological findings of focal brain radionecrosis. a Calcifications (HES, 60× magnification). b histiocytic inflammation (HES, 70× magnification). c Enlargement of Virchow-Robin spaces (HES, 70× magnification). d Gliosis in the adjacent white matter with dystrophic astrocytes (HES, 100× magnification)
The pathophysiology of focal brain radionecrosis is not well understood. Kamiryo et al. [46] followed changes in the rat brain within one year of radiation at doses of 50, 75, and 120 Gy mimicking therapeutic radiosurgery. The authors observed time- and dose-dependent changes. The first lesions to appear were morphological changes in astrocytes followed by vasodilation and fibrin deposition in capillary walls for all the studied doses. BBB leakage (Blue Evans permeability assay) was observed only after 75 and 120 Gy and necrosis only after 120 Gy.
An earlier study by Calvo et al. [47] focused on radiation-induced damage in the choroid plexus of the rat brain after a single dose of 17.5–25 Gy. They observed early morphological changes in the epithelial cells, followed by interstitial fibrosis associated with degenerative changes of arterioles and thrombi. Severity of lesions was dose and time-dependent. The same authors showed that necrosis was more frequent and occurred earlier in the fimbria than in the internal capsule and corpus callosum. They found a correlation between the incidence of necrosis in the white matter seen after a latent interval of 26 weeks and earlier changes in the vasculature such as blood vessel dilatation, blood vessel wall thickening, endothelial cell nuclear enlargement, and hypertrophy of perivascular astrocytes suggesting a continuous long-lasting mechanism induced by irradiation. Necrosis would be a consequence of ischemia secondary to blood vessel damage. At the molecular level, VEGF seems to play a pivotal role in the endothelial cell loss [48]. This progressive loss would eventually lead to overt necrosis [49]. Despite these findings, several points argue against a purely ischemic model: first, neurons are very sensitive to ischemia and should be prominently damaged if the lesion was primarily vascular whereas neurons are in fact largely spared during radionecrosis [50]. Second, vascular damage is not always present in radionecrosis [51]. Other cellular mechanisms may be involved and perpetuate vascular damage or contribute to edema, gliosis, and demyelination in the brain, such as upregulation of diverse adhesion molecules [52], production of cytokines [53, 54] or cumulative oxidative stress in endothelial cells.
Patients may experience seizures (first symptom in about 50% of cases), intracranial hypertension and/or focal neurological deficits [55–57]. Such symptoms closely mimic tumor recurrence or progression. Diagnosing radiation necrosis is often a challenge. In many cases, CT and MR scans show a tumor-like pattern, often indistinguishable from tumor progression or recurrence [58]. White matter FLAIR hyperintense lesions are the first imaging manifestation of focal brain radionecrosis. Contrast-enhanced lesions develop after white matter hyperintensities; their appearance changes with size, with an increasing tendency to necrosis with increasing size. Cysts are the least frequent and develop in the late stage of brain focal radionecrosis, always arising from a contrast-enhanced lesion that has undergone necrosis [59]. As diagnostic assessment based on clinical and standard neuroimaging data is difficult, many studies have tried to address the problem of noninvasive differentiation between tumor recurrence/progression and radionecrosis. Positron emission tomography (PET) with 18F-fluorodeoxyglucose [60] or 18F-Dopa [61] or 11C-methionine, single photon emission computed tomography (SPECT) with 201thallium or marked methoxy-isobutyl-isonitrile (99mTc-MIBI) [62] and the use of 3-[(123)I]iodo-alpha-methyl-l-tyrosine (IMT) [63, 64] have been used to assess the nature of the lesions. In typical cases, radionecrosis is characterized by hypometabolism and tumoral growth by hypermetabolism but results have been obtained on small series and standardization of detection thresholds is still awaited among teams [65]. The development of magnetic resonance spectroscopy (MRS) may help [66] as spectral analysis of necrosis areas shows an overall, harmonious decrease of metabolite peaks, and a possible increase of lipids corresponding to cellular necrosis; no lactate peak is observed [67, 68]. However, none of these techniques offers 100% sensitivity or specificity [69, 70]. One of the reasons for limitations is the frequent co-existence of radiation-induced necrosis with viable tumor tissue within the same area, a situation that obviously renders clear-cut distinction impossible [8, 65]. In some cases, angiography may provide further information, with an avascular mass seen in patients with radionecrosis; however, the risks and benefits of this procedure must be carefully weighed. While neuropathological examination remains the standard of diagnosis [71], even pathological analysis may be difficult because of the frequent mixture of both residual/recurrent tumor and radiation necrosis within the lesion [45].
Focal radionecrosis is sometimes spontaneously reversible even in the setting of a contrast-enhancing lesion [59]. Nevertheless, resection of a necrotic mass is often the best treatment in symptomatic cases. Steroids are generally used, with possible long-term improvement [8]; steroid dependence does occur. Other treatments have been reported in this setting, but their efficacy has not yet been addressed in large studies: anticoagulants have been prescribed by Glantz et al. [72] in 8 glioma patients (7 with histological evidence of necrosis) after the failure of steroids, leading to improvement in 5 patients. In our experience, anticoagulants have been somewhat disappointing. Some authors have advocated the use of hyperbaric oxygen (HBO) , with the rationale that HBO increases the tissue pO2 and enhances angiogenesis. Chuba et al. [73] treated 10 patients with CNS radionecrosis (biopsy-proven by biopsy in 8) with 100% oxygen for 90–120 min/session for at least 20 sessions. All patients stabilized or improved initially, and the 6 surviving patients showed durable improvement after 3–36 months. However, most patients were given steroids, and the respective effect of each treatment is not clear. HBO treatment was also reported to improve radionecrosis due to radiosurgery [74, 75]. The possible role of HBO in radiation-induced neurotoxicity needs to be evaluated in prospective trials [76]. Other drugs or combinations such as pentoxifylline, alpha-tocopherol [77], low-iron diet, desferrioxamine, and pentobarbital [1] have also been proposed occasionally without definite evidence of efficacy. The usefulness of radioprotective agents such as difluoromethylornithine (DMO) [78], U-74389G (a 21 aminosteroid) [79], or others [80–83], in reducing the risk of necrosis have been proved efficient in preclinical studies and request confirmation in clinical trials. There are increasing data about short-course bevacizumab therapy used for cerebral radiation necrosis, with symptomatic positive effects in case reports, small case series and one 14-patient randomized double-blind study [84]. Tye et al. [85] reviewed 16 publications from 2007 to 2012, including 71 cases where bevacizumab was administered for the treatment of focal brain radiation necrosis; 97% of the patients showed radiographic improvement and 79% improved performance status after treatment with bevacizumab. Bevacizumab is thus recommended in the second line setting following corticosteroids.
Cognitive Dysfunction and Leukoencephalopathy
Late-delayed cognitive impairment covers a wide continuum of patterns ranging from mild dysfunction to severe (and sometimes fatal) dementia, and has become an important concern over the last 20 years. The impact (and awareness) of this complication has grown because of both a better assessment of cognition and quality of life in clinical practice and the growing population of long-term survivors with tumor stability or remission.
Attributing all cognitive dysfunction to RT alone would result in a considerable overestimate of the incidence of radiation-induced sequelae. Cognitive impairment may be the consequence of complex interactions [3, 86, 87] between pre-existing cognitive abnormalities (especially in the case of brain tumors), brain tumor growth, concomitant treatments (such as chemotherapy, antiepileptic [88] or psychotropic drugs), paraneoplastic encephalomyelitis, and endocrine dysfunction. In practice, this should lead to an individualized workup in patients with potential radiation-induced cognitive impairment, but also to a careful and critical interpretation of the higher cognitive dysfunction rates found in the literature. Although high-dose per fraction (>2 Gy) is an important risk factor for induced cognitive impairments, decreases in cognitive function are also observed with small daily radiation doses [89].
Studies suggest that RT alone plays a more limited role in cognitive decline when using modern radiation techniques and fractionation schedules [27, 90, 91, 92]. Nevertheless, several factors have been clearly linked to an increased risk of radiation-induced cognitive impairment: (i) Advancing age at brain irradiation: several studies have demonstrated that demented patients were clearly older (55–60 years) than non-demented patients (<45 year old) [93–96]; (ii) Large radiation doses, particularly when fractions over 2 Gy are used; (iii) Large irradiated brain volume: this factor may also increase the risk of cognitive impairment; the rate of RT-induced cognitive dysfunction [94, 95, 97, 98] may be as high as 50% in patients receiving whole brain radiotherapy (WBRT) , whereas the precise incidence of cognitive impairment after focal brain radiotherapy is difficult to assess because of contradictory figures in the literature; (iv) Combined treatment: the incidence of dementia in patients treated with combined RT and chemotherapy ranges from 4 to 63%. Methotrexate (MTX) is clearly implicated in combined toxicity; neurotoxic by itself, it is responsible for frequent cognitive dysfunction when associated with RT. In children, most studies to date have concluded that the combination of cranial irradiation and intrathecal MTX was associated with declines in both IQ and achievement scores [99]. In adults treated with WBRT (40 Gy + 14 Gy boost) and a combination of intravenous and intrathecal MTX for CNS lymphoma, the incidence of severe progressive cognitive impairment increases with age, reaching 83% in patients over 60 years [96, 100, 101, 102]. The timing of MTX chemotherapy is important because the cognitive dysfunction rate is higher whenever MTX is prescribed during or after RT. This drug should therefore be given before irradiation. Data regarding the neurotoxicity of other combinations are sparse, but agents such as nitrosourea, cisplatin, etoposide, cytarabine, or actinomycin D are also suspected to increase radiation-induced cognitive toxicity. Multidrug regimens or high-dose chemotherapy combined with WBRT are probably associated with a higher risk of neurotoxicity [103]. Although there is a continuum between mild-to-moderate cognitive impairment and severe fatal dementia, we will consider the two conditions separately.
Radiation–Induced Mild–to–Moderate Cognitive Impairment
Mild-to-moderate cognitive dysfunction is more frequent in long-term survivors than frank dementia. The features of this condition are incompletely defined, as results vary across studies, likely attributable to neuropsychological evaluation procedures, duration of follow-up and population discrepancies [104]. Thus, the incidence of cognitive decrement related to radiation is difficult to evaluate. With anaplastic oligodendrogliomas and oligoastrocytomas, Habets et al. [92] reported that 30% of 32 progression-free patients were severely and 44% mildly impaired 2.5 years after initial chemoradiotherapy treatment. The most affected domains were psychomotor functions, attention and working memory, but these domains also manifested high interindividual variability. Further more, executive functioning and information speed processing were often weak, with results ranging from normal to pathologic. In another study, Douw et al. [89] explored the cognitive status of 65 low-grade glioma patients after a long-term follow-up (mean of 12 years, range 6–28 years) and observed that 53% of the patients who received radiotherapy were impaired in at least 5/18 neuropsychological test versus 27% in the patients who did not had radiotherapy. The most impaired functions were attention, executive functions, and information speed processing. Thus, radiation-induced cognitive impairment mainly affects attention, executive functioning, and information speed processing, while intellectual functions are generally preserved on neuropsychological evaluations [3, 86]. Nevertheless, most patients have to decrease or even discontinue their professional activities. These functions involve fontal corticosubcortical networks on which atrophy of white matter lesions can be observed. At this stage, CT scan may be abnormal, showing periventricular hypodensities, an increase in the normal interface between white and gray matter, and ventricular enlargement. However, there seems to be no correlation between CT scan abnormalities and the degree of cognitive impairment. MRI shows variable degrees of T2-weighted hyperintensities in the white matter but the functional correlate is not clear [24]. Fractional anisotropy may prove an interesting biomarker in normal-appearing white matter of patients treated with brain radiotherapy [105].
The course of radiation-related cognitive decline is difficult to predict: some patients deteriorate slowly while the majority apparently remain stable. Progression to dementia is seldom reported. There is no recognized treatment for this syndrome, although some authors have advocated the use of methylphenidate [106–108], anticholinesterase drugs [109, 110], memantine [111], or modafinil [108, 112, 113] for symptomatic relief. Psychological interventions may be useful [114, 115], as well as other non-pharmacological interventions like hyperbaric oxygen therapy [116], but solid replicated results are lacking. Day et al. [117] reviewed the randomized controlled trials that evaluated interventions aiming to reduce impairment related to cranial irradiation; they concluded there was a beneficial effect of donepezil and memantine despite limited studies.
Attempts to prevent cognitive impairment with potential neuroprotective agents or treatment methods have been made. Randomized trials have demonstrated that use of Motexafin Gadolinium (MGd) may protect against cognitive decline following whole brain radiation on cognition [118–120], though this effect may be related to this agent’s suppression of tumor growth. Phase 2 data suggest hippocampal sparing during radiation may prevent memory loss from fractionated radiotherapy for brain metastases [30, 121]. Sparing of other structures critical to cognitive functions (e.g., corpus callosum, frontal lobes, posterior fossa) might result in reducing neurotoxicity but unfortunately dose–response data are missing [122].
Other agents have been proposed for a potential neuroprotective effect, including erythropoietin (EPO) [123–125], lithium [126], and free-radical scavengers such as amifostine or angiotensin-converting enzyme inhibitors (ACEi) [127, 128].
Radiation–Induced Dementia
The incidence of this devastating complication varies widely in the literature (from 0% to more than 60%) according to the series. In a large review of several studies comprising 748 adult patients, the incidence of severe cognitive impairment compatible with dementia was at least 12.3% [129]. More recent studies and clinical practice are more reassuring [96, 130].
A “subcortical dementia” pattern that probably reflects the consequences of diffuse white matter injury characterizes the clinical picture.
The histopathological lesions of this radiation-induced leukoencephalopathy are similar to focal brain radionecrosis, consisting of well-demarcated areas of disseminated white matter necrosis (Fig. 14.5), myelin pallor and axonal alterations (which appear swollen and fragmented with calcifications), spongiosis, histiocytic inflammation, white matter gliosis, fibrotic thickening of small blood vessels in the deep white matter (Fig. 14.6), and atherosclerosis of the large vessels. Its pathogenesis remains unclear. Due to the difficulty in obtaining human tissue, histopathological studies of irradiated brains in human are scarce. However, a few autopsy studies in adults and children are available and show similar features of vascular and demyelinating lesions at light microscopic level. Lai et al. [131] studied the brain of 5 adult patients with primary CNS lymphoma who were in complete remission but who died after combined modality therapy with WBRT and chemotherapy. The MRI showed cerebral atrophy, ventricular dilatation, and white matter hyperintensity on FLAIR and T2 images. Occasional enhancing lesions were observed. The histopathological lesions were myelin and axonal loss, spongiosis, gliosis in white matter, fibrotic thickening of small blood vessels in the deep white matter and atherosclerosis of the large vessels in the circle of Willis. All patients but one were older than 60, and symptoms of neurotoxicity developed within 3 months of completion of treatment. Another autopsy study included 34 children with gliomas, 22 of whom had undergone CNS radiation therapy [132]. Causes of death were not detailed in this study and cognitive status of children before they died was unknown. Lesions such as demyelination, focal necrosis, cortical atrophy, endothelial proliferation, vascular thrombosis, vascular thickening were more frequently observed in irradiated brains, whereas neuronal degeneration, cerebral edema, and gliosis were common in both irradiated and unirradiated brains. Demyelination was observed at all time points from 6 months but was more common 9+ months after radiotherapy. Vascular changes were a late effect of radiation injury. Panagiotakos et al. [133] performed an original and extensive study on human normal and irradiated white matter brain samples from surgical biopsies of peritumoral glial tissue. Samples from irradiated patients exhibited persistent loss of oligoprogenitors starting as early as 2 months after radiation, whereas the decline of more mature oligodendrocytes only started beyond a year after irradiation. Early and transitory endothelial cell loss was noted. Myelin sheaths showed signs of degradation. Signs suggesting axonal damage were only seen long after irradiation. Neuronal cell bodies seemed to be spared from radiation injury.



Fig. 14.5
Radiation-induced leukoencephalopathy. Histopathological findings of radiation-induced leukoencephalopathy showing well-defined limitation between the areas of necrosis (asterisk) and preserved brain parenchyma (surrounded zone) (HES, 25× magnification)

Fig. 14.6
Radiation-induced leukoencephalopathy. Histopathological findings of radiation-induced leukoencephalopathy showing pallor of the white matter (asterisk) associated with gliosis and fibrotic thickening of small blood vessels (arrow) (HES, 80× magnification)
Because histological examination usually shows both vascular lesions and demyelination, vessels and glial cells have often been considered as the primary target of postirradiation leukoencephalopathy [134]. A short discussion of the main aspects of the pathophysiology of RT-related injuries follows.
Vascular Damage
Transient disruption of the blood–brain barrier, possibly initiated by sphingomyelinase-mediated endothelial apoptosis, is observed in animal models [45, 135, 136]. Thus, a cascade could be initiated at the time of irradiation, producing gradual cell loss throughout the clinically silent period. At the molecular level, VEGF seems to play a pivotal role in the endothelial cell loss [48]. This progressive loss would eventually lead to overt necrosis [49]. Despite these findings, several points argue against a purely ischemic model: first, neurons are very sensitive to ischemia and should be prominently damaged if the lesion was primarily vascular whereas neurons are in fact largely spared during radiation-induced leukoencephalopathy [50]. Second, vascular damage is not always present in animal models [51]. Other cellular mechanisms may be involved and perpetuate vascular damage or contribute to edema, gliosis, and demyelination in the brain, such as upregulation of diverse adhesion molecules [52], production of cytokines [53, 54] or cumulative oxidative stress in endothelial cells.
Oligodendrocytes
The demyelinating lesions observed after RT underscore the putative role of oligodendrocytes as a target of radiation damage. Oligodendrocytes are responsible for the production of the myelin sheath in the CNS and derive from progenitor cells such as O2A. CNS radiation induces depletion of oligodendrocytes and suppresses, at least transiently, the production of oligodendrocytes progenitors [137–139], possibly through a p53-dependent pathway [140–142]. However, the contribution of demyelination to tissue destruction remains questionable since severely demyelinating conditions such as multiple sclerosis do not lead to overt necrosis [50].
Other CNS Cell Types
Several studies have focused on the potential role of neurons, astrocytes, and microglia in the development of radiation-induced lesions, either as primary targets or through alterations of their regulatory capacity [143–145]. Particularly noteworthy is the possible role of microglia [146] that may enhance radiation injury through persistent oxidative stress [147]. Radiation damage to the subventricular zone, a tissue containing glial and neural stem cells, was reported more than 40 years ago [148]. Others studies have found a dose-dependent reduction of neural stem cells of the subependyma after irradiation in this region [149, 150]. Their implication in radiation injury thus seems probable but remains to be elucidated. As a whole, the development of radiation injury is complex, and results from interactions between vascular cells and oligodendrocytes, as well as neural stem cells and the physiological responses of these cells to injury.
Patients present with progressive memory and attention deficits, intellectual loss, gait abnormalities, emotional lability, apathy, and fatigue [151]. The absence of hallucinations or delirium and the very unusual. Occurrence of aphasia, agnosia, or apraxia (deficits suggesting cortical involvement) are important clinical features for narrowing the differential diagnosis, especially in elderly patients. Depression is frequent, but antidepressants do not improve cognitive function. Eventually, patients may develop gait ataxia, incontinence, and sometimes a picture of akinetic mutism. Nonspecific features such as seizures, pyramidal or extrapyramidal signs, or tremor are also frequently encountered in the course of the disease. Neuroimaging always shows diffuse white matter lesions, best seen on MRI as T2-weighted hyperintensities, associated with cortical and subcortical atrophy as well as ventricular enlargement (Fig. 14.7a–c). When performed, the lumbar puncture usually shows normal-to-moderately elevated (<1 g/l) CSF protein levels.


Fig. 14.7
a–c Radiationo-induced leukoencephalopathy. A 67-year-old patient treated with partial surgery followed by radiochemotherapy for a left parietal glioblastoma. MRI shows progressive signs of leukoencephalopathy with periventricular diffuse white mater hyperintensities and cortical atrophy. a Axial T2 FLAIR after surgery and before radiochemotherapy. b Axial T2 FLAIR 5 months after radiotherapy. c Axial T2 FLAIR 15 months after radiotherapy showing a progressive increase
No specific treatment is currently able to cure radiation-induced dementia. However, as the clinical features are similar to those of normal pressure hydrocephalus, some authors have advocated ventriculoperitoneal shunting; this procedure does improve the quality of life in a few selected patients [96, 152, 153]. In our experience, cognitive and gait-oriented rehabilitation are usually helpful.
Deterioration occurs in about 80% of cases, leading to death; stabilization is possible (18% of cases). Lasting improvement is exceptional. Death generally occurs within 1–48 months after the onset of the disorder [154].
Other Long-Term Direct Brain Parenchyma Radiation-Induced Lesions
Recent advances in high-grade gliomas treatment have increased the awareness that progressive lesions on MRI do not always mean tumor recurrence [18]. Importantly, the possibility of pseudoprogression is not entirely restricted to the early post-radiochemotherapy period [155].
It has also been suggested that post-radiation cortical vasculopathy might lead to the occurrence of non-epileptic reversible neurological symptoms, which have been described as the SMART syndrome (Stroke-like Migraine Attacks after Radiation Therapy) [156]. SMART syndrome is clinically defined by episodic cephalalgia with transient focal neurological deficit mimicking migraine aura years after radiation for brain tumor. Interestingly, these symptoms are associated with transient dramatic cortical gadolinium enhancement of the affected cerebral hemisphere [156, 157] (Fig. 14.8a–c).


Fig. 14.8
a–c SMART syndrome. 72 year old patient treated for a high-grade glioma with radiochemotherapy (30*2 Gy). 7 years after the treatment, the patient presented with transitory cephalalgias, motor deficit and right hemiparesis. a Axial T1. b Axial T1 with gadolinium injection showing contrast-enhancing nodular pericortical lesions. c Axial T2 FLAIR showing focal white mater hyperintensity
Approximately half of patients with brain tumors develop seizures during the course of their illness [158], and the occurrence of a first seizure or the recurrence of epilepsy after prolonged seizure control is frequently associated with tumor relapse. However, brain tumor-related seizures can also result in transient MRI abnormalities that can wrongly suggest tumor progression. This phenomenon has recently been described and called peri-ictal pseudoprogression (PIPG) [159].
Recently, 5 patients sharing some common features, including a remote history of whole brain irradiation in young to middle age, acute but long-lasting (4–24 days) impaired consciousness (Glasgow Coma Scale score 3–10), and clinical improvement after high-dose steroids were reported [160]. Vigilance impairment rapidly progressed to coma within 24–72 h. All patients required intermediate or intensive care. Other neurologic signs and symptoms included motor deficits and aphasia, headache, and visual hallucinations. Several consecutive EEG recordings performed during the acute phase showed bilateral/diffuse slow abnormalities in all patients, with unilateral predominance. MRI showed multiple bilateral areas of subcortical patchy enhancement and focal leptomeningeal enhancement. These were resolved at a follow-up MRI performed 5–8 months later. This syndrome was termed ALERT, for acute late onset leukoencephalopathy after radiotherapy.
Radiation-Induced Brain Tumors
The precise role of RT in the development of a tumor in any given case is difficult to determine, in great part because these tumors lack distinctive features compared to unirradiated patients. However, data from animal and epidemiological studies indicate that irradiated patients or animals are more likely to develop a second brain tumor than would be expected [161].
Several large studies have assessed the relative risk of developing a radiation-induced tumor. A study of 10,834 patients treated with low-dose cranial and cervical irradiation for Tinea capitis (mean dose to neural tissue: 1.5 Gy) found a relative risk of developing a tumor of 6.9; the risk for glioma was 2.6 [162].
In another study of 10,106 survivors of childhood cancers [163], the relative risk of developing a secondary CNS tumor was 7. Most other studies have found similar results [164, 165]. However, the risk is probably higher in patients treated for acute lymphoblastic leukemia (ALL) : a large retrospective cohort study of 9720 children [166] found a relative risk of developing a nervous system tumor as high as 22. In another setting, a large study on second brain tumors in 426 patients with pituitary adenoma treated with surgery and radiotherapy showed a 2.4% risk at 20 years [165].
Criteria for a radiation-induced tumor include: (a) long interval between radiotherapy and the occurrence of the second tumor (the mean onset delay is 12 years, with cases ranging from 1 to 40 years); (b) tumor growth within the radiation portal or at its margins; (c) a different histological subtype. After stereotactic radiosurgery, radiation-induced neoplasms are extremely rare, with only four reported cases [167]. Three types of tumors have been reported to be linked with cranial irradiation: meningioma in about 70% of cases, glioma in 20%, and sarcoma in fewer than 10%.
More than 900 cases [168] of radiation-induced meningiomas have been reported dating back to 1953 [169]; female predominance is less prominent than in spontaneous meningiomas [170]. The risk of occurrence correlates with radiation dose: low-dose RT induced a relative risk of 9.5 in one study [162], whereas high-dose RT was linked to a relative risk of 37 [164]. The tumor emerges after a long latency period: a review found extremes ranging from 2 to 63 years (mean 18.7 years) after high-dose RT [171].
Histopathologically, the most frequent subtypes are meningothelial and transitional (Fig. 14.9a–c), followed by fibroblastic and angioblastic [172], but other meningioma variants have been described after irradiation including metaplastic meningiomas (xanthomatous, myxomatous) [173, 174], chordoid meningiomas [175].


Fig. 14.9
a–c Radiation-induced meningiomas. Histopathological findings of a case of radiation-induced meningioma. a High cellular proliferation compound of meningothelial cells with small foci of necrosis (asterisk) (HES, 130× magnification). b Pleomorphic tumoral cells with prominent nucleoli and mitosis (arrow) (HES, 300× magnification). c High MIB-1 labeling index (120× magnification)
In the majority of cases, radiation-associated meningiomas are benign, multiple, and recurrent (World Health Organization grade I) [168, 176]. Nevertheless, atypical (grade II) or anaplastic (grade III) meningiomas have been also frequently reported. They evidence features of malignancy (Fig. 14.10a, b) such as nuclear pleomorphism with great variation in nuclear size, shape and chromatin density, high cellularity, increased mitotic figures, atypia and necrotic changes [168, 172, 177, 178] and high MIB-1 labeling index. Other nonspecific findings include numerous multinucleated and giant cells, thickened blood vessels and vacuolated nuclear inclusions [168]. Radiation-induced meningiomas are sporadic.
Cytogenetic studies have been performed in some radiation-induced meningiomas and showed numerous genetic alterations such as loss of 1p and 7p chromosomes [179], loss of 22q12.2 [175], loss or deletion on choromosome 6 [180]. Indeed, these chromosomal alterations evoked by radiation accelerate loss of cellular control mechanisms and earlier expression of the neoplastic phenotype [181, 182]. Radiation-induced gliomas are much less frequent. Since 1960, about 120 cases have been reported in the English literature [183, 184]; fewer than half of these were glioblastomas. In the group of patients treated with RT for acute leukemia, multifocality occurred in 20% of cases. The median delay of onset ranges from 6 to 9 years [184]. It is well recognized that radiation-induced gliomas in children are high grade [185]. Among these radiation-induced gliomas, all histopathological subtypes have been reported: high-grade tumors such as glioblastoma, anaplastic astrocytoma or oligodendroglioma (Fig. 14.10a, b), but more rarely low-grade tumors such as grade II diffuse astrocytomas [186]. Gliosarcoma , characterized by mixed glial and mesenchymal elements (Fig. 14.11a–c), is a classical radiation-induced glioma and may result from the sarcomatous transformation of an irradiated glioma [187, 188]. The tumorigenesis of radiation-induced gliomas seems to be multifactorial, resulting from the impairment of cellular immunity, immunosuppressive effects of radiation and direct cellular effects of this treatment [189]. Few series have studied molecular changes in radiation-induced gliomas. It appears these tumors manifest similar alterations of PTEN, EGFR, and TP53 alterations to sporadic gliomas [190] but do not depend upon classical molecular pathway implicated in gliomagenesis such as IDH1/2 mutations [191], except when they result from the sarcomatous transformation of an irradiated glioma [187]. Moreover, most of the reported cases of malignant transformation of pilocytic astrocytomas (WHO grade I) received prior radiation therapy [192].



Fig. 14.10
a, b Radiation-induced oligodendroglioma. Histopathological findings of a case of radiation-induced oligodendroglioma with signs of malignity. a High cellular proliferation with endothelial proliferation (arrows) and necrosis (asterisk) (HES, 50× magnification). b Oligodendroglial proliferation with pleomorphism, atypia, and numerous mitoses (arrows) (HES, 200× magnification)

Fig. 14.11
a–c Radiation-induced gliosarcoma. Histopathological findings of a case of radiation-induced gliosarcoma. a High cellular proliferation compound of pleomorphic cells with a spindle cell component (HES, 90× magnification). b GFAP immunoreactivity in a subset of tumor cells (180× magnification). c Expression of vimentin in spindle tumor cells (130× magnification)
The prognosis of these tumors is poor: intrinsic resistance to treatment as well as previously received aggressive therapies substantially limit available therapies.
Fewer than 40 cases of sarcomas have been reported to date, including several histological types (e.g., meningiosarcomas, neurofibrosarcomas) [161].
Radiation-Induced Vasculopathy
Distinct from radionecrosis, in which severe lesions of the arterioles and capillaries constitute a cardinal feature, radiation (particularly of tumors near the central arterial circulation) can induce other types of vascular damage leading to stroke or intracerebral hemorrhage and must be considered as comorbidity predictive of stroke [193].
Large and Medium Intra- and Extracranial Artery Injury
An arteriopathy affecting the large cervical blood vessels, especially the carotid artery [194], is a recognized complication of cervical radiation therapy, usually administered for lymphomas or head and neck cancers. Intracranial vessels may also be affected. The main early-delayed vascular complication is carotid rupture [195, 196], which usually follows a few weeks after cervical RT and surgery for head and neck tumors. Associated skin lesions such as necrosis or wound infection are common. The outcome of this exceptional complication is very poor.
Late-delayed complications are more frequent, and generally occur many years after RT (median time approximately 20 years for extracranial, 7 years for intracranial artery lesions). The lesions are similar to those induced by atherosclerosis, but are often located in unusual places for common atherosclerosis and occur in an accelerated fashion. It has been observed that the larger the diameter of an irradiated artery, the longer the latency between RT and the onset of vasculopathy, a fact that might explain the shorter latency of RT-induced vasculopathy in children. Shorter latencies have also been reported with interstitial radiotherapy [197]. The dose required to induce vascular lesions usually exceeds 50 Gy, but the type of radiation, fractionation, and portal differs greatly from one case to another. The lesions consist of one or more stenoses or occlusions in the arteries included within the radiation portal. The diagnosis, suspected when a cervical murmur is heard in the immediate vicinity of radiation-induced skin lesions, relies upon magnetic resonance angiography, ultrasound examination, and arteriography. The treatment is similar to that of usual atherosclerotic lesions; in the event of carotid stenoses, endarterectomy may be appropriate. However, surgery may be more difficult than in unirradiated patients because of vascular fibrosis and skin lesions, with higher postoperative risk of infection or wound healing problems. In other patients, antiplatelet agents may be prescribed if there is no contraindication. Some authors have advocated lowering serum cholesterol levels to prevent the development of such lesions in patients at risk [193, 194, 198].
Radiation-Induced Vasculopathy with Moyamoya Pattern
Intracranial vasculopathy leading to a progressive occlusive disease and a moyamoya pattern (characterized by abnormal anastomoses and netlike blood vessels) accounting for focal seizures, strokes, or transient ischemic attacks may follow intracranial irradiation, especially in very young children. This complication is particularly frequent in children treated for optic chiasm glioma, a condition often associated with neurofibromatosis type 1 (NF-1, which is a risk factor for vasculopathy itself). It may also occur with other tumors such as brainstem glioma and craniopharyngioma [199]. In a series [200] of 69 childrens (11 with NF-1) treated for optic pathway glioma with RT (median dose 55 Gy), 13 (19%) developed clinical and radiological signs of vasculopathy after a median latency of 36 months. The strong association between NF-1 and moyamoya is one of the reasons why radiation has been replaced with chemotherapy in younger children [130]. The treatment focuses on preventing further strokes through surgical revascularization techniques; calcium blockers such as flunarizine have been advocated by some authors [201–204]. The role of antiplatelet agents has not been defined in this setting.
Silent Lacunar Lesions
A report [205] described a rare pattern of silent cerebral lacunes occurring in children treated for brain tumors. In this study reviewing 524 consecutive children, 5 of 421 treated with RT and chemotherapy had lacunes. Median age at tumor diagnosis and RT administration was 4.5 years old, and lacunes developed after a median latency of 2 years (ranging from 0.26 to 6 years). This pattern was associated with no further clinical deficit or neuropsychological impairment when compared to patients without lacunes. This condition is probably linked to delayed radiation-induced capillary and small vessel lesions.
Radiation-Induced Cavernomas , Angiomatous Malformations , and Aneurysms
Brain vascular malformations such as telangiectasiae and cavernomas [170, 206, 207] have been rarely observed following RT (Fig. 14.12a–c). Ocular telangiectasiae may also occur [208]. When present, their main risk is intracranial bleeding. Several cases of multiple radiation-induced cavernous angiomas have also been reported [209], occurring 18 months to 23 years after RT.


Fig. 14.12
a–c Radiation-induced cavernomas. A 46-year-old patient treated with chemotherapy and radiotherapy 22 years previously for an anaplastic astrocytoma. MRI shows cavernomas in the cerebellum. a Axial SWAN sequence showing 2 cerebellar carvernomas. b Axial T1 with gadolinium injection. c Axial T2 FLAIR
Histopathologically, radiation-induced cavernomas do not differ from sporadic or familial cavernomas (Fig. 14.13a–c), and are characterized by juxtaposition of abnormal vessels showing thick walls and conspicuous fibrin deposits [210]. This vascular malformation is often accompanied by gliosis, numerous macrophages and calcifications [210]. The pathogenesis of radiation-induced cavernomas remains unclear. Indeed, those cavernomas may exist before radiation and hemorrhage in response to the radiation [207, 211].


Fig. 14.13
a–c Radiation-induced cavernoma. Histopathological findings in a case of radiation-induced cavernoma. a Juxtaposition of abnormal vessels showing thick walls (arrows) (HES, 50× magnification). b Calcifications (asterisk) (HES, 50× magnification). c Expression of CD34 by endothelial cells (50× magnification)
Finally, fewer than 15 cases of radiation-induced intracranial aneurysms have been described in the literature [212, 213]. The median age of the patients was 37.5 years (ranging from 11 to 65 years) with a latency of 10 months to 21 years; there was no correlation between the onset of aneurysm and the radiation dose. This represents a rare but severe problem, as rupture is always possible; 6 of 9 aneurysmal ruptures proved fatal. An enlarging aneurysm can also mimic tumor recurrence. Aneurysms are sometimes detected preclinically with standard imaging procedures for tumors (CT scan and MRI) [214], and particular attention should be drawn to evaluating the onset of such lesions during imaging follow-up. When an aneurysm is detected on CT or MR scan, or if the clinical history strongly suggests its presence, cerebral angiography is required for delineation.
Endocrine Dysfunction
Frequently underestimated [130, 131, 215], endocrine disorders can be the consequence of direct irradiation of a gland (e.g., the thyroid gland, with about 50% of patients developing hypothyroidism within 20 years following radiotherapy for Hodgkin disease or certain head and neck cancers) or result from hypothalamic–pituitary dysfunction secondary to cranial irradiation (several authors believe that the hypothalamus is more radiosensitive than the pituitary gland) [216]. We will focus on the second type of disorder, which can be induced by cerebral or nasopharyngeal tumor irradiation.
There is a positive correlation between radiation dose and the incidence of endocrine complications. In a prospective study on 268 patients treated with different brain RT schemes Littley and colleagues found that the incidence of TSH deficiency was 9% after treatment with 20 Gy, 22% with 35–37 Gy, and 52% with 42–45 Gy 5 years after RT [217]. Hormonal deficits can appear at any time after RT but may arise more rapidly in patients treated with higher radiation doses [218].
In children, varied endocrine deficits may result from cranial RT (administered for brain tumors or during prophylactic irradiation in acute lymphoblastic leukemia). Growth hormone (GH) is usually the first and in many cases the only anterior pituitary deficit in young patients. This complication affects approximately 50% of children treated with prophylactic cranial RT for acute lymphoblastic leukemia [219].
According to a Danish study of 73 children treated with RT for a primary brain tumor (not involving the hypothalamo-pituitary axis directly) and with a long follow-up (median 15 years), 80% of patients manifested growth hormone deficiency; the median biological effective dose (BED) in the hypothalamo-pituitary area was higher in GH-deficient children than in patients without GH deficiency [220]. Administration of GH is recommended in children with growth hormone deficiency; however, as GH has no effect on vertebral bodies, long-term survivors acquire a typical “spiderlike” physical appearance with long extremities and short trunk [130]. Subtle central hypothyroidism is common in children and should be treated with thyroxine replacement therapy in order to limit the potential for thyroid carcinoma as well as to improve longitudinal growth [221, 222].
In adults, a study [223] evaluating 31 long-term brain tumor survivors followed 1.5–11 years after RT with a mean total dose of 62.3 Gy compared with 31 matched controls found hypothalamic hypothyroidism in 26% of patients, hypothalamic hypogonadism in 32% of patients, hyperprolactinemia in 29% of patients and panhypopituitarism in one patient. Low adrenal hormone levels were found in most patients, but without apparent clinical consequence. In the control group, only 6% had a baseline hormonal concentration outside the normal range. None of the controls had two or more hormonal abnormalities, while 42% of the patients had multiple deficits. Only 23% of patients had normal thyroid, gonadal and adrenal baseline levels; this result is consistent with an earlier study reporting hypothalamic–pituitary dysfunction in 10 of 13 (77%) long-term survivors irradiated for supratentorial low-grade glioma [224]. Another study of patients treated for nasopharyngeal cancer found secondary hypothyroidism in 27% of cases (of hypothalamic origin in 19% and pituitary origin in 8%) [225].
The neurological consequences of severe hypothyroidism are well known, including encephalopathy, cerebellar ataxia, pseudomyotonia, and sometimes peripheral neuropathy. Increased CSF protein is also usual. All these abnormalities may be misleading if the correct diagnosis is not considered.
Secondary hypogonadism is an important concern, especially in male patients, responsible for decreased libido and sometimes impotence impacting negatively on quality of life. Hyperprolactinemia of hypothalamic origin is a notable concern in women who develop oligomenorrhea and galactorrhea [226]; in men, it may result in gynecomastia and decreased libido.
Follow-up consultations provide an opportunity for regular clinical endocrine evaluations; the precise biological follow-up scheme is debated and is adapted according to the emerging deficits, but long-term assessment should be the rule. The treatment of hormonal deficits lies in replacement therapy, and usually leads to an improvement in the patient’s condition. Bromocriptine has been utilized with success in patients with symptomatic hyperprolactinemia [161].
Sequelae of Radiotherapy to the Spinal Cord
Radiation for the treatment of spinal cord tumors, Hodgkin disease, mediastinal, or head and neck cancers may eventuate in spinal cord damage. Early descriptions in the 1940s [227] were followed by numerous descriptions of post-radiation myelopathy delineating the main clinical patterns: early-delayed myelopathy and several types of late-delayed complications including progressive myelopathy, lower motor neuron disorder, and spinal hemorrhage. There is no clear clinical or experimental evidence of acute spinal cord toxicity due to RT, and a sudden worsening during radiation should lead to a search for intratumoral hemorrhage or tumor progression [8].
Early-Delayed (Transient) Radiation Myelopathy
The onset of this complication occurs from 6 weeks to 6 months after RT, and improvement follows in most cases within 2–9 months [228], though persistence of the symptoms for a longer time is possible in rare cases. It usually follows radiation to the cervical or thoracic spinal cord. After mantle RT for Hodgkin disease, early-delayed myelopathy occurs in 15% of cases [229]. Another study reported a global incidence of 3.6% (40 cases among 1112 patients receiving 30 Gy or more). The incidence was 8% in patients receiving 50 Gy or more, 3% after doses of 45–49.9 Gy, 4% after doses of 40–44.9 Gy, and 2% after doses of 30–39.9 Gy. The risk was also increased with a fraction size >2 Gy [230].
The clinical pattern first described by Esik et al. [231] generally consists of Lhermitte’s phenomenon triggered by neck flexion, and is characterized by brief unpleasant sensations of numbness, tingling, and/or often electric-like feelings from the neck to the spine and extremities. No MRI abnormalities are associated with this condition. This symptom is nonspecific, and other causes should be considered in a patient with cancer [232] including chemotherapy (cisplatin or docetaxel), spinal tumor, vitamin B12 deficiency, herpes zoster, or even multiple sclerosis (which may be aggravated by radiation).
The presumed pathophysiology of early-delayed myelopathy is transient demyelination, probably secondary to a loss of oligodendroglial cells following RT [233, 234]. There is no specific treatment for this condition, and none is required, as recovery occurs in most cases. Early-delayed radiation myelopathy is not predictive of evolution to the much more serious progressive myelopathy.
Late-Delayed Radiation-Induced Spinal Cord Disorders
Spinal radionecrosis (Fig. 14.14a–c) (with features similar to its cerebral counterpart), progressive myelopathy, and spinal hemorrhage have been described as late complications of spinal radiation.


Fig. 14.14
a–c Spinal radionecrosis. 52 year old patient treated with radiochemotherapy (40 Gy in 16 fractions) 29 years earlier for Hodgkin deases. a Axial T1 with fat saturation showing intramedullary hyperintensity. b Sagittal T1. c Sagittal T1 gadolinium showing contrast enhancement
Progressive Myelopathy , or Delayed Radiation Myelopathy (DRM)
This complication occurs 6 months to 10 years after exposure to RT. Risk factors include advancing age, large radiation doses and fractions, previous irradiation especially in childhood, and large portals involving thoracic or lumbar spinal cord [228]. Chemotherapy may increase the risk of delayed radiation myelopathy [235]. The generally accepted tolerance for the spinal cord is 45 Gy in 22–25 daily fractions, with a risk <1% for a dose of 50 Gy increasing to 5% for a dose of 60 Gy delivered in 1.8–2 Gy fractions [236, 237].
No histological study in humans has been reported. Radiation of the rat spinal cord leads to progressive abnormalities in the white matter beginning at 19 weeks for doses greater than 17 Gy. The spinal cord of paralyzed animals showed areas of necrosis and demyelination, but neither gross vascular lesion nor inflammatory infiltrate was found [48]. Occasional vessel dilation was observed.
Delayed radiation myelopathy may begin abruptly or, more often, in a progressive way; patients complain of sensory and/or motor deficits leading to para- or tetraparesis. A typical initial clinical presentation is a Brown–Sequard syndrome, consisting of a motor deficit associated with ipsilateral sensory loss affecting tactile, vibration, and passive movement sense on one side, and contralateral sensory loss affecting temperature and pain sensory modalities. In some patients, a transverse myelopathy develops with bilateral leg weakness and sensory loss up to the irradiated region. Some patients also experience pain. Bladder and bowel sphincter as well as diaphragmatic dysfunction (in upper cervical spinal cord lesions) are possible. The evolution of delayed radiation myelopathy varies; in some patients the symptoms stabilize, while in others they progress to a complete deficit.
The diagnosis of delayed radiation myelopathy implies—as was underlined as early as 1961 by Pallis et al. [238]—that the site of the main lesion is within the radiation-exposed area of the spinal cord and that all other potential causes of myelopathy have been carefully reviewed and eliminated.
Spinal cord MRI is helpful, though nonspecific. The initial description of Wang and colleagues [239, 240] has been confirmed in several subsequent studies [241–243]: the initial MRI may be normal if performed during the first weeks of the disease, but a slightly delayed examination usually reveals a swollen cord with T1-weighted hypointensity and T2-weighted hyperintensity. Lesions enhance in about 50% of cases after gadolinium injection (Fig. 14.14a–c) [244, 245]. In contrast, late examinations, performed years after the onset of the disease, may show spinal cord atrophy without any signal abnormality; a case of cystic formation in late-delayed radiation myelopathy has also been reported. Moderately elevated protein is the most common finding in the CSF but lacks any specificity. If performed, somatosensory evoked potentials show changes correlated to the extent of the lesions, whereas spinal conduction velocity is decreased.
Corticosteroids may improve some patients, probably because of their action on the inflammatory and edematous components of the disorder; however, patients often become steroid-dependent and only a few experience long-term improvements. There is no current proven long-term treatment for delayed radiation myelopathy. However, Angibaud and colleagues reported the efficacy of hyperbaric oxygen in stabilizing or improving 6 out of 9 patients with DRM [246], and Calabro and workers reported a similar case [247]. Anticoagulation has also been tried, with improvement in one patient with myelopathy treated for >3 months with full anticoagulation and stabilization in another treated with coumarin [72]. Two case reports suggested that bevacizumab might be of benefit [248] in radiation-induced myelopathy.
Late-Delayed Spinal Hematoma
This rare complication has only been described in a few cases, following spinal radiotherapy by 6–30 years, and occurring within the radiation portal but outside the location of the primary tumor [249]; acute-onset leg weakness and back pain rapidly lead to para- or tetraparesis. The diagnosis relies on MRI demonstration of hemorrhage.
Spontaneous symptom resolution is possible, but new episodes may occur later. There is no proven effective treatment for this condition. Avoidance of aspirin or nonsteroid anti-inflammatory agents is prudent. Radiation-induced telangiectasias with secondary hemorrhage may be the culprit.
Sequelae of Radiotherapy on the Cranial Nerves
Apart from acute reversible radiation toxicity, all cranial nerves may be involved in late-delayed radiation-induced complications if included in the radiation portal (usually during the treatment of neck and head tumors). These complications are rare, probably arising in fewer than 5% of cases after conventional radiotherapy (70, 2 Gy per daily fraction) [250]. Modern intensity modulation radiation therapy protocols likely reduce this rate further [251]. Cranial nerve dysfunction occurs during the first five years post-radiation therapy [251]. Large daily radiation fractions increase this risk, which can reach 47% with a median follow-up of 7.6 years with a median equivalent dose in 2 Gy fractions of 94 Gy in nasophayngeal carcinoma patients [252]. Thus radiosurgery also puts cranial nerves at risk. Based upon results of a phase III study including nasopharyngeal carcinoma patients, concomitant chemotherapy has also been described as a risk factor of cranial nerve toxicity [250]. Moreover, a study in brain tumor survivors found that 17% of patients developed neurosensory impairment and that RT exposure greater than 50 Gy to the posterior fossa was associated with a higher likelihood of developing any hearing impairment [253]. The principal complications are described below for each cranial nerve.
Olfactory Nerve Injury
Olfactory dysfunction is rare but classically reported with radiotherapy [254–256]. This may be due to direct acute stimulation of the olfactory neurons. Anosmia has also been described in some patients [257], often associated with taste disorders [258].
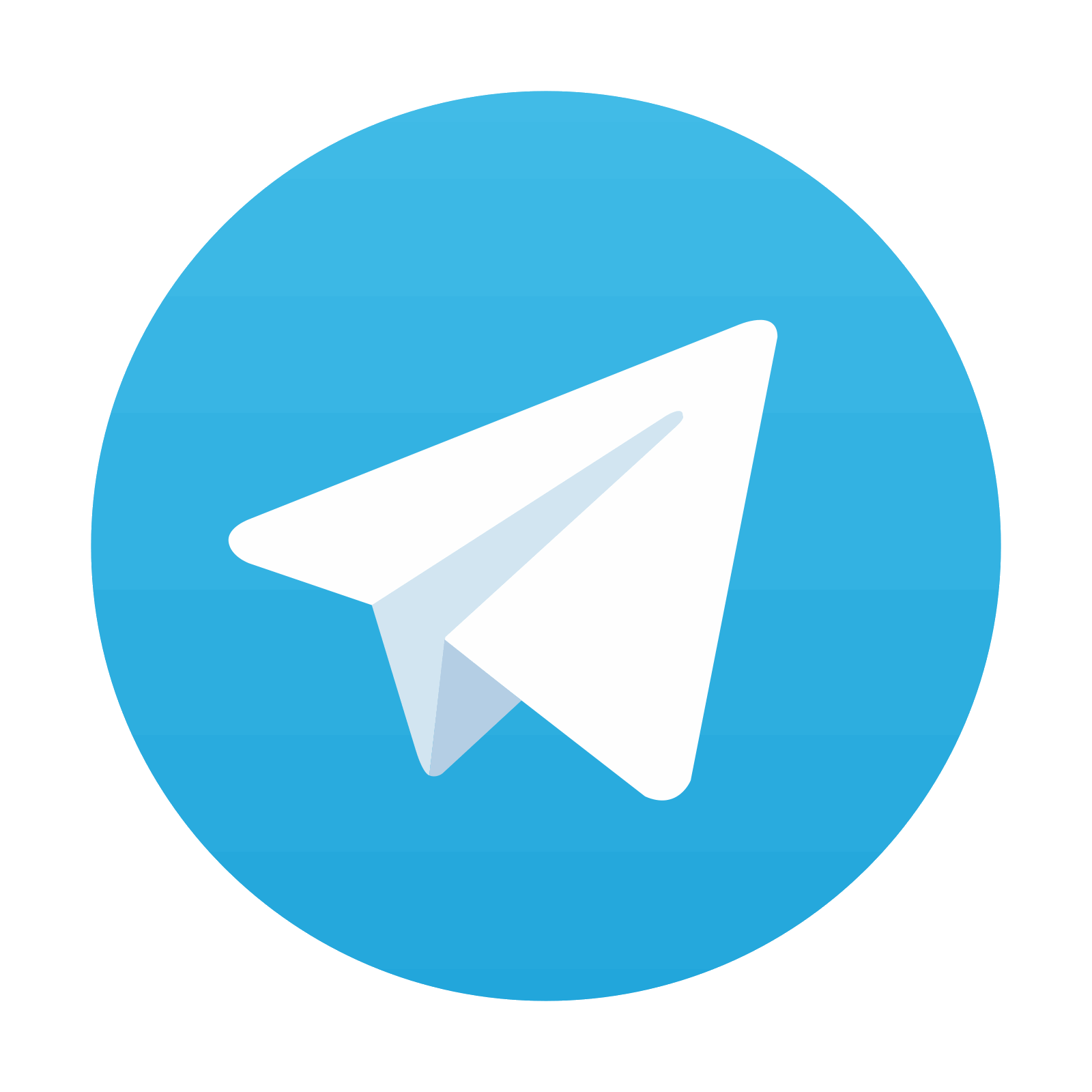
Stay updated, free articles. Join our Telegram channel
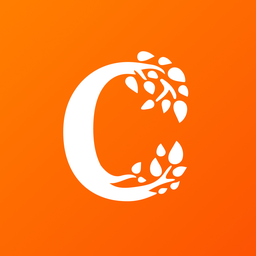
Full access? Get Clinical Tree
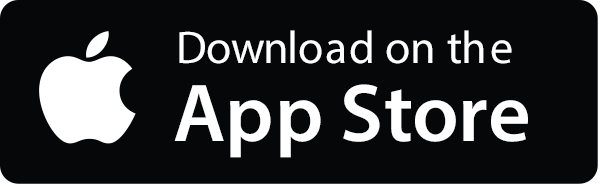
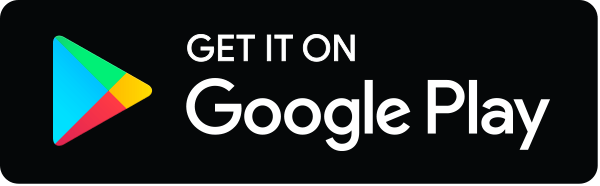