Fig. 21.1
A right frontal non-enhancing glioma with increased T2 (left) and FLAIR (right) signal abnormality. It can be difficult to distinguish vasogenic edema from infiltrative tumor in a case such as this
Blood–Brain Barrier and Edemagenesis
The pathogenesis of vasogenic brain edema involves disruption of the blood–brain barrier. This physiologic barrier separates the systemic circulation from the central nervous system (CNS) and consists of the specialized endothelium of blood vessels supplying the CNS surrounded by pericytes. This barrier normally impedes the entry of most water-soluble but not lipid-soluble agents [8, 9]. The anatomic features essential for the normal function of the blood–brain barrier are highlighted in Fig. 21.2 [10].


Fig. 21.2
Normal blood–brain barrier demonstrating tight junctions between endothelial cells; a normal basement membrane and adjacent astrocyte foot processes (used with permission of Cambridge University Press from Francis et al. [10])
Disruption of the blood–brain barrier by a brain tumor results in increased entry of water-soluble substances and macromolecules such as plasma protein into the tumor and surrounding brain. Because there is no lymphatic system in the CNS, these substances are not easily eliminated, and are driven into surrounding brain tissue by increased hydrostatic pressure within the tumor, resulting in vasogenic brain edema. The edema tends to extend along white matter tracts rather than in the more closely packed gray matter [11].
The mechanisms of blood–brain barrier disruption and formation of brain edema are incompletely understood, and multiple factors are involved. When brain tumors grow to a size greater than 1–2 mm, they promote formation of new blood vessels that lack a normal blood–brain barrier. This tumor-associated vascular endothelium is characterized by an increased number of intercellular junctions, discontinuous tight junctions, membranous fenestrations, non-contiguous basement membranes, and active micropinocytosis. This results in increased permeability of these new blood vessels to macromolecules, ions, and proteins, with the formation of vasogenic edema. All of these microvascular abnormalities are most common in the central portion of the tumor and least common at the interface between brain and tumor [12–16]. Vascular endothelial growth factor (VEGF) , secreted by tumor cells, may be responsible for the disruption of the blood–brain barrier in the tumor as well as the peritumoral region [17]. There is also evidence that arachidonic acid and its leukotriene metabolites promote vasogenic edema formation by selectively increasing capillary permeability within the tumor. Arachidonic acid is a normal constituent of membrane phospholipids in the CNS but may be released into the extracellular space under certain pathologic conditions [18–21]. Finally, immunologic mechanisms may be important, as tumor growth is associated with macrophage infiltration in and around the tumor. These inflammatory cells are capable of elaborating a variety of secretory factors that are associated with increased vascular permeability [6]. One study demonstrated that the amount of tumor-associated edema visible on CT scans correlated with the extent of macrophage infiltration found on pathologic study [22]. The relative contribution of each of these mechanisms to edemagenesis is unknown.
Blood–brain barrier disruption and the subsequent development of vasogenic edema lead to increased intracranial pressure (ICP). Because the process is caused by a focal brain lesion, the increased intracranial pressure is distributed unevenly within the intracranial compartment and may result in brain herniation and compromise of local blood supply [23]. These events contribute to the neurological symptoms and signs observed in patients with increased ICP secondary to brain tumors.
Steroid Therapy
Glucocorticoids are the mainstay of treatment for vasogenic brain edema. Kofman and colleagues [24] were the first to demonstrate the responsiveness of brain tumor edema to corticosteroids, and Galicich and French [25] introduced dexamethasone therapy as the standard treatment for tumor-associated edema. The mechanism of action of corticosteroids in the treatment of vasogenic brain edema is incompletely understood [26]. A mechanism commonly thought to account for the beneficial effects of corticosteroids is a direct action on endothelial cell function which restores normal vascular permeability [27, 28]. One possible mechanism by which corticosteroids may restore normal permeability involves the ability of corticosteroids to inhibit the release of arachidonic acid, a substance known to increase vascular permeability and promote formation of brain edema [29–31]. It has been shown that corticosteroids can have both direct and indirect effects on endothelial cells and may inhibit increased permeability by interfering with the ability of the cell to interact with permeability factors [8, 31]. It is also known that corticosteroids reduce the filtration of plasma-derived fluid across tumor capillaries and reduce the movement of albumin through the extracellular space by solvent drag [32]. Finally, there is indirect evidence that dexamethasone causes cerebral vasoconstriction [33]. Likely, all of these mechanisms contribute to the ability of corticosteroids to stabilize the blood–brain barrier and lead to reduction of vasogenic brain edema. Using diffusion tensor MR imaging, Sinha and colleagues have demonstrated that administration of corticosteroids decreases peritumoral extracellular water content in edematous brain but does not affect the water content of contralateral normal brain [34]. This finding was seen in cerebral metastases, gliomas, and extra-axial tumors.
The optimal dose of corticosteroids for vasogenic brain edema has not been established. Dexamethasone is commonly used in clinical practice and is probably the best steroid for the treatment of brain tumors [8]. The advantages of dexamethasone include the absence of mineralocorticoid effect, which makes salt retention and systemic edema less likely. There is also evidence that of all the corticosteroids, dexamethasone is less likely to be associated with infection and cognitive impairment [30, 35]. Nevertheless, like other fluorinated corticosteroids, dexamethasone is more likely to cause myopathy [36].
Since corticosteroids cause adverse side effects and induce metabolism of other drugs, the benefits of corticosteroids in patients with asymptomatic brain edema are generally outweighed by these side effects. Alternatively, patients with symptomatic brain edema typically respond and, therefore, usually benefit from the use of corticosteroids. The patient should be maintained on the lowest dose that controls neurological symptoms in order to avoid the development of adverse effects. The drug is absorbed from the gastrointestinal tract, but first-pass hepatic metabolism may decrease effectiveness, especially in patients also receiving phenytoin [37, 38]. Dexamethasone can be given twice daily because of the longer half-life of this steroid, although clinicians sometimes prescribe it 4 times daily [39]. A commonly used starting schedule is a 10-mg oral dose followed by 4 mg 4 times a day, which is 20 times the rate of endogenous cortisol production; however, the dosage should be tailored to the individual patient’s clinical and imaging characteristics [30]. There is evidence that doses lower than 16 mg daily may be just as effective with the same degree of clinical improvement after 1 week of treatment [39]. Approximately 70–80% of patients with brain tumors will experience improvement of symptoms with dexamethasone [8, 40]. A rapid clinical response to steroid administration indicates that the symptoms may be primarily due to the tumor-associated edema rather than actual tumor mass [30]. Symptoms and signs of generalized brain dysfunction such as headache and lethargy respond more rapidly and dramatically to corticosteroids than focal neurological signs. If the standard dose fails to achieve a clinical response in 48 h, then the dose can be doubled every 48 h until response occurs. Rarely, up to 100 mg of dexamethasone over 24 h may be required in occasional patients; however, the advent of bevacizumab has led to an alternative to corticosteroids as discussed below [41, 42].
In patients with brain tumors, symptomatic improvement usually begins within hours of an intravenous injection of corticosteroids. Positron emission tomography (PET) scans of humans with brain tumors demonstrate an effect on the blood–brain barrier as soon as 6 h after the intravenous bolus [43]. Maximal clinical improvement usually occurs within 24–72 h. It has been shown that the first change is a decrease in plateau waves followed by a gradual decline of increased intracranial pressure over a period of 48–70 h [44]. Improvement on CT and MR imaging studies may lag behind clinical improvement, although early scans may show decreased contrast enhancement within the tumor, suggesting partial restoration of the blood–brain barrier [27, 45, 46].
In order to avoid the deleterious effects of corticosteroids, the patient should be treated with the smallest effective dose for the shortest time possible [47]. The patient should be tapered from corticosteroids during or after more definitive treatment such as surgery, radiation therapy, or chemotherapy. The taper should generally start 3–4 days after surgery and during the second week of radiation therapy. In general, patients with malignant brain tumors should receive standard dose corticosteroids for 48–72 h prior to starting brain irradiation to reduce intracranial pressure and minimize acute radiation toxicity. For patients on a standard dose of 16 mg of dexamethasone per day, decreasing the dose by 2–4 mg every fifth day is reasonable. If symptoms of steroid withdrawal or increased brain edema occur, the drug should be increased to the immediately preceding level for 4–8 days before starting the taper again. If symptoms of brain edema recur after discontinuation, the dexamethasone may need to be resumed to control symptoms if alternative strategies are not available [8]. A more rapid taper may be used safely to minimize steroid toxicity, decreasing the dose from 16 mg/d for 4 days to 8 mg/d for 4 days followed by 4 mg/d until completion of radiation therapy [48]. In patients on corticosteroids for many months who fail the usual taper schedule or in patients with a large amount of residual tumor, the drug is tapered more slowly (e.g., 1–2 mg/wk) to the lowest dose possible. Patients on large doses of corticosteroids (e.g., 100 mg of dexamethasone per day) who have stabilized and are receiving definitive treatment can have the dose halved every 4–5 days depending on the clinical condition [8]. As discussed below, the advent of bevacizumab has provided an alternative to corticosteroid therapy.
Deleterious Steroid Effects
The classic features of excessive corticosteroids, including weight gain, moon facies, acne, hirsutism, and abdominal striae, are easily recognized by most clinicians but rarely produce significant morbidity. Adverse effects that are potentially more serious include myopathy, cognitive impairment, gastrointestinal dysfunction, and opportunistic infection [8, 11].
There are conflicting reports on the frequency of steroid toxicity in patients with brain tumors [42, 49–51]. Duration of treatment appears to be an important variable with prolonged treatment (>3 weeks) associated with greater toxicity [47]. Hypoalbuminemia also appears to confer greater risk of steroid toxicity as the percentage of unbound steroid increases, especially with serum albumin levels less than 2.5 g/dL [47, 52, 53]. Because patients with brain tumors are often debilitated, they may be particularly susceptible to the deleterious effects of corticosteroids.
Most patients treated with conventional doses of corticosteroids (e.g., 16 mg of dexamethasone per day) for more than 2–3 weeks will develop some degree of myopathy. In one study, 15 cancer patients being treated with dexamethasone were followed for the development of myopathy. Within 15 days, nine patients had developed myopathy, which, in most, was sufficient to interfere with activities of daily living. The cumulative dose ranged from 186 to 1846 mg. The development of myopathy correlated with the total dose rather than the duration or daily dose of dexamethasone [54]. In steroid-induced myopathy, muscle biopsy shows atrophy of type II fibers, the fibers characterized by high glycolytic and low oxidative capacity [55]. Serum muscle enzymes are not elevated. Steroid myopathy is characterized clinically by proximal muscle weakness and eventual muscle wasting, especially in the pelvic girdle. One of the most common complaints is an inability to arise from the seated position. The myopathy may progress to involve the proximal arms and neck. Evaluation of neck and hip flexor strength usually provides the most sensitive clinical assessment for steroid myopathy [56]. It has also been recognized that respiratory muscle may be affected, and this can result in symptomatic dyspnea in severely myopathic patients [57]. Treatment includes reduction or discontinuation of corticosteroids, if possible. Since fluorinated corticosteroids (dexamethasone, triamcinolone) are associated with more type IIb fiber atrophy than non-fluorinated corticosteroids (prednisolone, methylprednisolone) avoidance of the former may lower the risk of steroid myopathy, although this has not been studied systematically. Preclinical experimental observations have suggested that alternate-day dosing of methylprednisolone reduces the severity of myopathy compared to continuous daily dosing of the same drug [58]. Exercise, physiotherapy, and a high-protein diet during steroid treatment may attenuate the disorder [59]. One study reported a decreased frequency of myopathy in brain tumor patients treated with corticosteroids who also received phenytoin, potentially due to increased catabolism of the steroid after induction of the hepatic microsomal system by phenytoin [37].
Psychiatric complications may develop in as many as 5% of patients receiving exogenous corticosteroids. The majority of these are either affective or psychotic reactions, but other complications include delirium and neuropsychological impairment with selective involvement of attention, concentration, and memory [60, 61]. A prior history of an adverse psychiatric reaction to corticosteroids does not predict such future complication, and corticosteroids should not be withheld if medically indicated in such patients. Most patients with psychiatric complications make a full recovery, but symptomatic treatment may be necessary. Steroid reduction and neuroleptic administration are usually effective for psychotic symptoms [60]. One report suggests that lithium prophylaxis lessens the likelihood of a psychotic reaction to corticosteroids, although it is not routinely recommended [62]. Steroid withdrawal can also lead to depressive symptoms [63].
The major gastrointestinal effects of corticosteroids are ulceration and perforation [64, 65], although upper gastrointestinal bleeding is rare in patients taking corticosteroids with no previous history of such bleeding. The incidence is much higher with simultaneous use of anticoagulants or in patients with a prior history of upper gastrointestinal bleeding, although the overall risk in one study was less than 1% for such patients if treated with corticosteroids for less than 1 month [66]. In a study of nearly 46,000 patients using corticosteroids, Nielsen and colleagues found the relative risk of hospitalizations due to gastrointestinal bleeding was 4.9 compared to expected. The relative risk fell to 2.9 among patients using corticosteroids alone, without the use of other drugs known to cause gastrointestinal bleeding (e.g., aspirin) [67]. Whether or not patients on corticosteroids benefit from receiving gastrointestinal prophylaxis with antacids, H2-blockers, proton pump inhibitors, or other anti-ulcer agents remains controversial [8, 68]. Bowel perforation is a serious complication in steroid-treated patients and is associated with high mortality. It usually occurs in patients treated with high doses of corticosteroids who have been constipated as a result of medication, immobility, or neurological dysfunction. The perforation usually affects the sigmoid colon and may not be accompanied by the usual abdominal symptoms and signs due to the masking effects of corticosteroids or comorbid neurological disease. This usually delays diagnosis and may account for the high mortality rate in this group of patients [69, 70]. Plain radiographs usually are diagnostic, and surgical repair remains the definitive treatment. The goal, however, should be prevention of this complication with careful attention and treatment of constipation, including adequate bulk in the diet, hydration, stool softeners, and laxatives as necessary.
Steroids are immunosuppressive drugs. In one study, 24% of primary brain tumor patients receiving concurrent corticosteroids and radiation experienced a reduction in their CD4+ cell count to <200 cells/mm3 [71, 72]. Opportunistic infections secondary to immunosuppression from corticosteroids include Candida mucositis and esophagitis as well as Pneumocystis jiroveci pneumonia (PJP). The rate of PJP in patients with brain tumors is increasing, and studies have demonstrated incidence rates of 1–6% for this group of patients. Most of these patients were also receiving corticosteroids for prolonged periods, and infection was most likely to occur during the steroid taper [73–76]. Trimethoprim–sulfamethoxazole given as a single double-strength tablet either daily or thrice weekly during steroid administration and for 1 additional month afterward reduces the incidence of PJP by approximately 85% and should be considered in patients with brain tumors who are likely to require prolonged steroid treatment [73, 77].
Osteoporosis is a common complication of prolonged steroid use. While most patients with brain tumors do not live long enough for this to lead to fractures, it is important to recognize that steroid-induced osteoporosis can be prevented and treatments instituted, as appropriate, to the individual patient [78, 79]. Calcium in combination with vitamin D may prevent bone loss [80]. There are several recent studies that demonstrate the efficacy of biphosphonates, such as alendronate or risedronate, in the prevention of osteoporosis in patients taking chronic corticosteroids [81–83].
Another bone complication of steroid use is avascular necrosis of the hip or other bones which may develop following prolonged use of corticosteroids or may occur after only a few weeks of therapy [84–86].
Lipomatosis is the result of chronic steroid use, which stimulates redistribution of fat. Deposition of fat may occur in the epidural space and result in spinal cord compression. MR imaging is usually diagnostic, and surgical treatment may be necessary [87–89].
Visual problems consist mainly of blurring, which is a common complaint and is probably due to a change in refraction caused by corticosteroids. This usually improves with reduction or discontinuation of the corticosteroids. Longer-term steroid use may result in glaucoma or cataract formation [8].
Other possible side effects include hyperglycemia, which occurred in 19% of neuro-oncology patients receiving corticosteroids in one survey [47]. The majority of such patients required insulin or modification of the steroid dose to control hyperglycemia in another study [50]. Transient anogenital burning or tingling may occur with intravenous administration of dexamethasone and can be distressing if the patient is not warned of such a possibility [90]. Hiccups, nocturia, and diminished sense of smell and taste have also been reported as complications of corticosteroids [8, 91, 92].
Another potential hazard of steroid use is the development of a withdrawal syndrome during the steroid taper. Steroid pseudorheumatism is the most common withdrawal syndrome and is heralded by the onset of diffuse arthralgias and myalgias mimicking rheumatoid arthritis. These symptoms may be debilitating, but there are usually few physical findings. Either reintroduction of low-dose corticosteroids followed by a slower taper or treatment with aspirin or other nonsteroidal anti-inflammatory agents may result in improvement [11]. Amatruda and colleagues [93] described a steroid withdrawal syndrome which may include lethargy, headache, dizziness, anorexia, and nausea. These symptoms may confuse the clinician by suggesting worsening of the underlying brain tumor [11].
Due to the fact that dexamethasone results in the potent induction of specific cytochrome P450 (CYP450) isozymes (CYP3A4; CYP2C8; CYP2C9), there is a potential for significant drug interactions with other agents metabolized by this system.
Bevacizumab Therapy
Bevacizumab is a recombinant humanized monoclonal antibody that binds all isoforms of vascular endothelial growth factor (VEGF) [94], a protein which is central to vascular proliferation and permeability. While the precise mechanism is not yet known, competing theories on the action of bevacizumab include either direct suppression of angiogenesis or normaliziation of dysmorphic vessels within tumors [95].
With bevacizumab therapy, MR imaging is often rapidly and dramatically changed, and interpretation thereof may be challenging. Whereas anti-angiogenic therapy targets the vasculature, the blood–brain barrier is altered, as well as gadolinium and fluid extravasation. As such, contrast enhancement and peritumoral FLAIR hyperintensity decrease on MRI, yet tumors may still progress in a non-enhancing fashion [96].
While bevacizumab received an accelerated approval for recurrent glioblastoma in 2009 [97] based on two positive phase II trials [98], subsequent large phase III studies of bevacizumab in newly diagnosed glioblastoma failed to demonstrate overall survival advantage over standard therapy alone [99, 100]. Nonetheless, many clinicians still use bevacizumab for glioblastoma, not only for the benefit of progression-free survival (which was borne out in the aforementioned trials), but also for the purpose of reducing tumor-associated edema. While the latter has not been studied directly in trials, a surrogate marker of edema reduction was reported in several clinical trials where average dose of corticosteroids was decreased in bevacizumab-treated patients. In general practice, many neuro-oncologists use bevacizumab for the reduction of neurological symptoms from brain tumors and brain tumor-associated edema.
On the whole, bevacizumab is felt to have a reduced side effect profile when compared to corticosteroids, though still may cause adverse events, some life-threatening. Potential adverse events include both intra- and extracrial hemorrhage, thromboembolic events, hypertension, proteinuria, wound dehiscence, and others. For a more complete discussion of bevacizumab adverse events, refer to the chapter on complications of targeted agents, Chap. 16.
Emergency Therapy of Brain Edema and Increased Intracranial Pressure
Hyperventilation
Immediate treatment of brain edema and increased intracranial pressure (ICP) may occasionally be necessary to prevent death or cerebral herniation. The methods available for such treatment are outlined in Table 21.1. Hyperventilation is the most rapidly effective technique available for decreasing ICP. Hyperventilation decreases the partial pressure of carbon dioxide (pCO2) which causes cerebral vasoconstriction in undamaged areas of the brain and a consequent decrease in cerebral blood volume and ICP. Intracranial pressure decreases within 30 s of lowering pCO2 and remains low for 15–20 min but usually returns to the original level by 1 h [101]. Usually, the patient is intubated and is ventilated to decrease the pCO2 to 25–30 mm Hg. The patient must be monitored carefully, as mechanical ventilation may occasionally increase ICP and patients with brain lesions are especially susceptible to this effect [102].
Table 21.1
Emergency treatment of cerebral herniation
Therapy | Dosage or procedure | Onset (duration) of action |
---|---|---|
Hyperventilation (minutes) | Lower pCO2 to 25–30 mm Hg | Seconds |
Osmotherapy | Mannitol, 0.5–2.0 g/kg (IV) over 15 min followed by 25-g booster doses (IV) as needed | Minutes (hours) |
Corticosteroids | Dexamethasone 100 mg IV push followed by 40–100 mg every 24 h depending on symptoms | Hours (days) |
Osmotherapy
The mechanism by which hyperosmolar agents lower ICP remains a matter of dispute [103, 104]. At least part of the explanation is the ability of these agents to create an osmotic gradient between the blood and that part of the brain with an intact blood–brain barrier driving the movement of water from the extracellular space to the site of the higher osmolarity in the blood. The agent most commonly used for osmotherapy is mannitol, which is usually given as 20–25% solution in a 0.5- to 2.0-g/kg intravenous bolus over 10–20 min. Mannitol is effective within minutes, and the effect is sustained for several hours [105–107]. If there is clinical worsening after initial improvement, smaller intravenous boluses of mannitol may be administered, but repeated doses of mannitol may cause a rebound increase in ICP, especially in patients with vasogenic brain edema [30]. There is also some evidence to suggest that the combination of mannitol and a loop diuretic such as furosemide produces a more significant and sustained decline in ICP, although furosemide has no role in the chronic management of tumor-related edema [108]. Varying concentrations of intravenous sodium chloride (e.g. hypertonic saline) are also used to reduce intracranial pressure through osmotic gradient formation.
High-Dose Steroid Therapy
In patients with cerebral herniation, plateau waves, or signs of increased ICP, an intravenous bolus of dexamethasone (e.g., 40–100 mg) followed by doses of 40–100 mg/d may be effective in reversing brain herniation [8]. The addition of furosemide (40–120 mg intravenously) to the steroid dose may be better than corticosteroids alone [109]. Similarly, bevacizumab may be used to reduce cerebral edema causing raised intracranial pressure. Other available methods of lowering ICP such as barbiturate anesthesia and hypothermia are reviewed in detail elsewhere [106]. With the above-mentioned emergency therapies, most patients herniating from the effects of a brain tumor stabilize and improve.
Seizures
Epidemiology and Pathogenesis
Seizures are common in patients with primary brain tumors. The frequency of epilepsy varies with the tumor type. Vertosick and colleagues reported that >80% of patients with low-grade gliomas have epilepsy [110], whereas the reported prevalence of epilepsy in glioblastoma ranges from 30 to 50% [111], Lieu and colleagues reported epilepsy in 40% of meningiomas [112], and Hochberg and colleagues reported epilepsy in 20% of patients with primary CNS lymphoma [113].
In a series of patients receiving chemotherapy for supratentorial tumors (nearly all gliomas), Hildebrand and colleagues found that 78% of 234 patients had epilepsy [114]. In 86% of the patients with epilepsy, the epilepsy was an early manifestation of the disease and usually the presenting manifestation. In only 14% did the epilepsy begin with malignant transformation of the glioma. Seizures were clinically characterized as focal, focal with secondary generalization and generalized. Focal seizures alone were more common late in the course of the disease.
The cause for this high rate of seizures in brain tumor patients may be related to neoplastic astrocytes. While normal astrocytes take up extracellular glutamate, glioma cells have been reported to release excitotoxic levels of glutamate [115, 116], which has been hypothesized as a mechanism for tissue invasion and genesis of seizures [117, 118]. Furthermore, this report indicates that the anticonvulsants valproate, phenytoin, and gabapentin decreased the calcium-mediated glutamate release by astrocytes and decreased seizures as well. Whether glutamate antagonists could have a clinical anti-neoplastic role as demonstrated in animal models by Takano and coworkers [117], or serve as an antiepileptic for patients with brain tumors, is unknown.
Since seizures are associated with increased CNS blood flow, they may significantly increase ICP and potentially lead to a herniation syndrome. Furthermore, seizures in patients with primary brain tumors are more likely to result in a post-ictal neurological deficit (Todd’s paralysis) [8, 119, 120]. Status epilepticus is rare in patients with brain tumors but can occur and has an associated mortality of 6–35% [8, 121].
Symptomatic Treatment
Since patients with brain tumors and seizures have a high risk of recurrence of seizures, treatment of these patients with AEDs is warranted. The selection of a particular anticonvulsant requires consideration of individual patient and drug factors as well as the other types of therapy the patient is receiving. Many of the older anticonvulsants either induce cytochrome P450 (CYP450) enzymes (phenytoin, carbamazepine, oxcarbamazepine, phenobarbital) or inhibit CYP450 enzymes (valproic acid). Thus, there is a potential for significant drug interactions or altered metabolism in brain tumor patients who are receiving anti-neoplastic drugs metabolized by the same enzymes. In fact, changes in the plasma levels of chemotherapeutic drugs that could be clinically significant have been observed (Table 21.2) [122–126]. When available, blood levels of AEDs should be monitored, as many patients with brain tumors are also receiving other medications such as dexamethasone which may cause AED levels to fluctuate.
Table 21.2
Influence of enzyme-inducing anti-seizure drugs on the total body clearance of intravenously administered chemotherapeutic agents in cancer patients
Anticancer agent | Infusion time (h) | Dose (mg/m2) | Total body clearancea (l/h/m2) | Difference (%) | Ref. | ||
---|---|---|---|---|---|---|---|
−EIASD | +EIASD | −EIASD | +EIASD | ||||
Etoposide | 6.0 | 320–500 | 320–500 | 0.80 | 1.42 | 76.9 | 110 |
Irinotecan | 1.5 | 112–125 | 411 | 18.8 | 29.7 | 58.0 | 111 |
Paclitaxel | 3.0 | 240 | 240 | 4.76 | 9.75 | 104.8 | 112 |
Teniposide | 4.0 | 200 | 200 | 0.78 | 1.92 | 146.2 | 113 |
Topotecan | 0.5 | 2.0 | 2.0 | 20.8 | 30.6 | 47.1 | 112 |
Vincristineb | 0.25 | 2.0 | 2.0 | 34.1 | 55.5 | 62.6 | 114 |
Since many of the newer AEDs (gabapentin, lacosamide, lamotrigine, levetiracetam, pregabalin, tiagibine, topiramate, zonisamide) do not induce the CYP450 system, these drugs are attractive options for brain tumor patients. To date, few studies have been performed to evaluate the efficacy and safety of these newer agents in patients with brain tumors. Levetiracetam is the most studied with three prospective, non-randomized studies demonstrating efficacy and acceptable safety, with the most notable adverse events of cognitive and psychiatric effects [127–129]. Further, Lim and colleagues randomized 29 patients treated with phenytoin to either continue phenytoin or switch to levetiracetam after craniotomy, and found that switching to levetiracetam was safer and resulted in fewer (but not significantly) seizures [130]. Only one randomized trial of non-enzyme-inducing seizure medications has been performed to date by Rossetti and colleagues, evaluating the use of either levetiracetam or pregabalin for the treatment of brain tumor-related epilepsy [131]. In this non-comparative, open-label study, the authors found that both levetiracetam and pregabalin were efficacious and safe for brain tumor-related epilepsy.
One controversial line of retrospective study is also worth mentioning: There may be a potential survival advantage of brain tumor patients whose seizures are treated with various AEDs. Weller and colleagues performed a subgroup analysis of the phase III European Organization for Research and Treatment of Cancer (EORTC) study of temozolomide for glioblastoma and reported an overall survival advantage with the use of valproic acid. However, this was an unplanned and underpowered subgroup analysis [132]. A retrospective analysis of subjects in the Nort Central Cancer Treatment Group suggested that treatment with enzyme-inducing seizure medications correlated with prolonged survival [133]. Additional prospective work is needed to better understand the interaction between AEDs and survival of brain tumor patients.
Treatment of gliomas with anti-neoplastic therapy can reduce the incidence of seizures. In an EORTC trial of early versus delayed radiotherapy in the management of low-grade gliomas, 25% of patients who had received early radiotherapy had seizures at one year versus 41% for those who did not receive early radiotherapy (p = 0.03); the prevalence of seizures was the same at diagnosis [134]. Hildebrand and coworkers reported that 56% were on monotherapy, 28% were on a combination of two AEDs, and 12% were on three AEDs. Additionally, there has been a report of a patient with an oligodendroglioma-induced epilepsy that was refractory to 14 anticonvulsants but responded to temozolomide [135].
Prophylactic Treatment
Although as noted above, many patients with primary brain tumors have seizures, the question often arises as to whether primary brain tumor patients without seizures should be placed on prophylactic AEDs. A meta-analysis of four randomized trials of seizure prophylaxis revealed no evidence of reduction in the frequency of first seizures in patients receiving prophylactic anticonvulsants [136]. As such, the potential benefit of prophylactic AED therapy with the studied drugs may not outweigh the risk of side effects in these patients, which include those commonly seen in any seizure patient, as well as some unique or far more common and serious in primary brain tumor patients. On the whole, available data suggest that brain tumor patients experience a higher frequency of AED complications (20–40% of patients) compared to the general population [136]. In six studies reporting AED side effects, 24% (5–38%) of brain tumor patients experienced adverse effects severe enough to warrant discontinuation of the drug. Complications included rash (14%); nausea or vomiting (5%); encephalopathy (5%); myelosuppression (3%); ataxia, increased liver enzymes or gum pain (5%) [136, 137]. A side effect worthy of special mention in patients with brain tumors is the Stevens–Johnson syndrome, which has been reported in patients on phenytoin and, less frequently, carbamazepine who are also receiving cranial radiation while on a decreasing dose of corticosteroids [6]. An additional consideration against prophylactic AED therapy is the interaction of certain AEDs with the CYP450 system and metabolism of chemotherapeutic agents, as discussed above. Finally, Klein and coworkers reported that AEDs were associated with six times the risk of reduced psychomotor speed and attention/executive dysfunction in patients who had undergone focal radiotherapy [138].
Based on the overall analysis of insufficient first-seizure reduction compared to potential side effects, the American Academy of Neurology (AAN) published a practice parameter in 2000 advising against the use of prophylactic AEDs. However, many clinicians still report treating brain tumor patients with prophylactic AEDs. In a survey of physicians in one state, 55% of the neurologists and 81% of the neurosurgeons prescribed prophylactic AEDs to brain tumor patients [139]. Since more clinically tolerable AEDs with less drug interaction have come into practice, no subsequent survey has been performed.
Perioperative seizure prophylaxis following craniotomy for tumor resection is generally considered as a separate category from long-term seizure prophylaxis in brain tumor patients. A prospective, randomized trial evaluated the risk of perioperative seizure either with or without prophylactic phenytoin therapy. Whereas the risk of seizures was quite low in both groups, the authors suggested that prophylactic phenytoin may not be necessary [140]. Further, two retrospective studies compared the reported incidence of perioperative seizures on levetiracetam prophylaxis versus the expected incidence without prophylactic AED, finding that perioperative levetiracetam prophylaxis was safe and effective [141, 142]. Finally, in a head-to-head comparison, Iuchi and colleagues prospectively randomized patients to either levetiracetam or phenytoin and found that levetiracetam lowered the incidence of postoperative seizures significantly more so than phenytoin [143]. In addition, the AAN has also issued a guideline that it is appropriate to taper and discontinue anticonvulsants after the first postoperative week in brain tumor patients who have not had a seizure, who are medically stable, and who are experiencing anticonvulsant-related side effects [136].
Non-convulsive Status Epilepticus
It is well established that brain tumors can cause seizures and non-convulsive status epilepticus. A single-institution review identified that, of 259 patients with an ICD-9 brain tumor diagnosis who underwent electroencephalogram (EEG), 2% were diagnosed with non-convulsive status epilepticus [144]. Treatment resolved the non-convulsive status epilepticus in 92% of those individuals, with accompanying clinical improvement in 75% of patients. Notably, the EEG may not always be helpful in establishing a definitive diagnosis of non-convulsive status epilepticus, which can only be made at times if the patient awakens following the administration of AEDs [145]. Interestingly, it has also been shown that non-convulsive status epilepticus can cause transient abnormal contrast-enhancing cortical lesions that could be confused with a brain tumor [146]. This information reinforces the fact that clinicians should maintain a high suspicion for non-convulsive status epilepticus in brain tumor patients with altered mental status, as this may represent a reversible cause.
Cognitive Effects of Brain Tumors
One of the hallmarks of brain tumors is cognitive dysfunction, which may be either acute and temporary or chronic and progressive. Cognitive dysfunction may result from the tumor itself, tumor-directed therapy, delirium, non-convulsive status epilepticus, medications, depression, or fatigue. Interestingly, in addition to location-specific dysfunction of a primary brain tumor and its therapies, the biology of the tumor itself may play a role in cognitive decline. A recent study revealed glioma grade to be an independent predictor of cognitive performance for certain locations of tumor, without regard to therapies delivered [147].
The potential neurotoxicity and cognitive effect of anti-tumor therapy is of great consideration when treating patients. This is especially true in patients with low-grade gliomas, who have a relatively longer prognosis. Reports are mixed on the cognitive effects of surgery, mostly based on small and observational studies. While some reports have demonstrated decline in short-term perioperative cognitive function, including domains such as memory, attention, and naming after surgery in eloquently located gliomas [148], others have suggested no such hazard [149]. Longer-term follow-up after surgical resection in eloquent areas is clearer, though, and overall suggests improvement in verbal memory after surgery for low-grade gliomas [150, 151].
Data are also sparse for the cognitive effects of chemotherapy for primary brain tumors. Prabhu et al. [152] evaluated the cognitive effects of adding procarbazine, lomustine, and vincristine chemotherapy to radiotherapy in a large, randomized trial among patients with low-grade gliomas. Performing the Mini-Mental State Examination (MMSE) at various intervals until five years post-therapy, they found no significant difference between patients receiving radiation alone or radiation followed by chemotherapy. Further, both groups had a significant improvement in MMSE over time. Among glioblastoma patients treated with temozolomide both concurrent and adjuvant to cranial irradiation, cognitive performance was stable after six months of therapy for those with stable tumors [153]. For treatment with bevacizumab, data are mixed. In the two large, phase III randomized trials evaluating the addition of bevacizumab to up-front glioblastoma therapy, neurocognitive findings were contradictory between trials [99, 100], with greater deterioration reported in patients treated with bevacizumab in RTOG 0825 than in AVAglio. Laboratory research has shown that bevacizumab may reduce the synaptic plasticity in hippocampi, elucidating a potential mechanism of cognitive decline with this drug [154]. Consensus guidelines now exist for further research on cognitive impairment related to chemotherapy for patients with all cancers [155].
Information about cognitive function and radiation therapy is clearer and more robust; cognitive deficits are one of the most common delayed-onset adverse effect of radiation [156]. Patients receiving brain radiotherapy commonly complain of fatigue in the hours following each treatment. Brain edema commonly develops subacutely following brain radiotherapy, especially in the case of stereotactic radiosurgery [157]. Later, patients suffer with various neurocognitive deficits at a far greater rate than similar patients without radiotherapy [158, 159]. These may occur reversibly weeks to months after radiation, or irreversibly and progressively months to years after radiation. In adults who receive radiotherapy, the most common domains to be affected include executive function, information processing speed, novel problem solving, verbal and spatial memory, and attention [159–161]. In survivors of childhood brain tumors who receive radiation, the greatest concern is long-term intelligence quotient impairment and impaired learning [162]. Notably, recent studies of proton therapy for the treatment of childhood brain tumors, in lieu of more common photon therapy, showed no evidence for cognitive decline [163, 164]. Further research on this radiation source is under way.
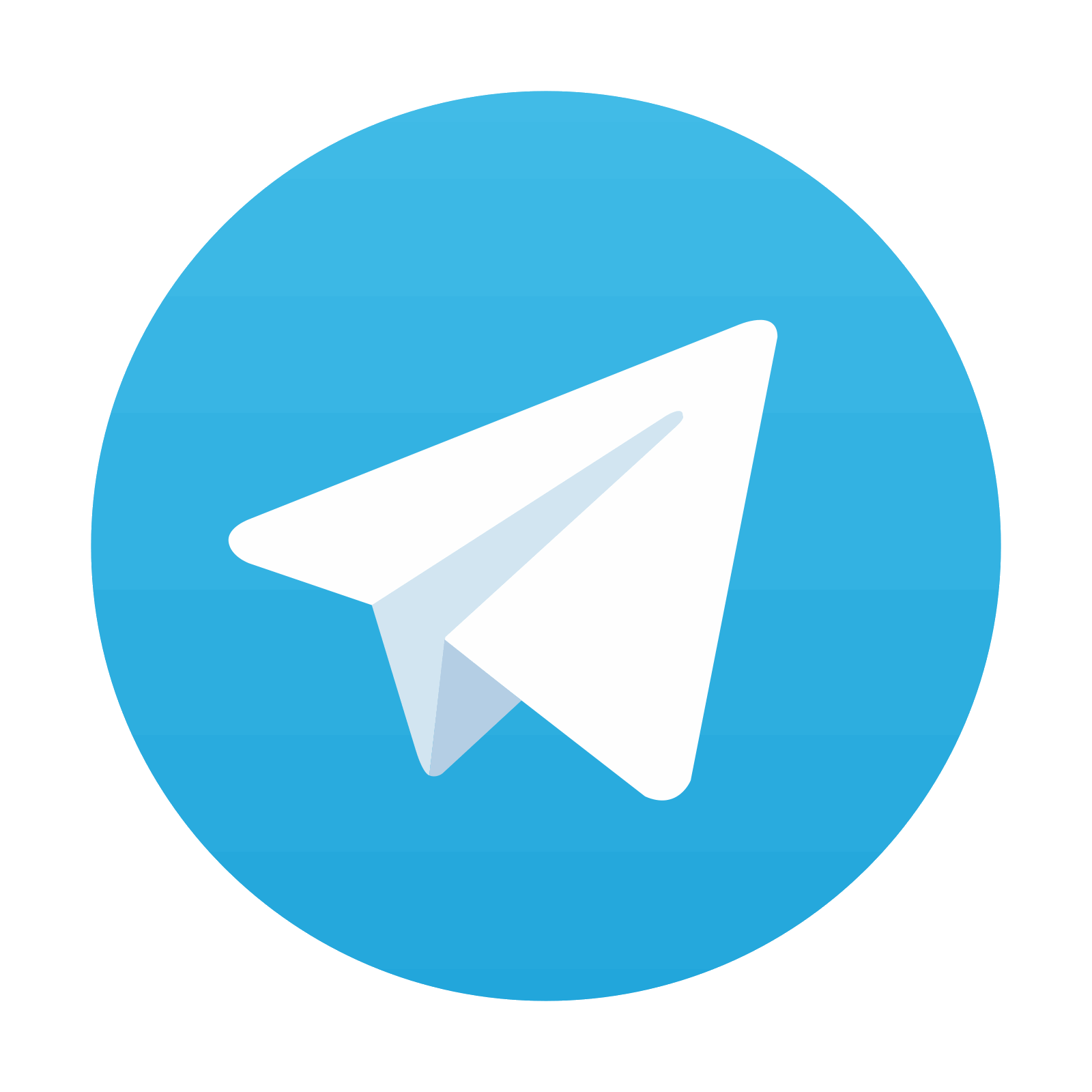
Stay updated, free articles. Join our Telegram channel
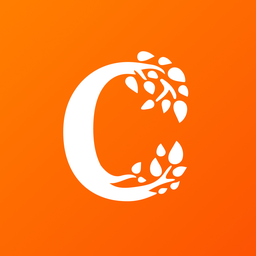
Full access? Get Clinical Tree
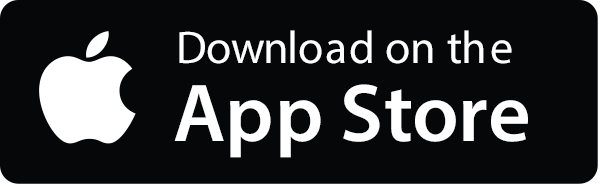
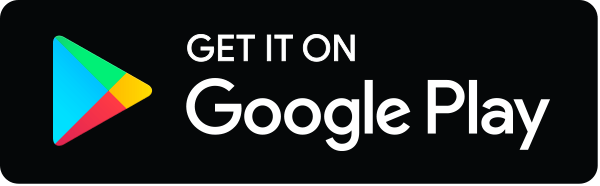