Introduction
The rapid pace of development in modern neuromodulation belies the ancient roots of such technology. Scribonius Largus, the Roman court physician, was among the first to describe the relief of pain following incidental contact with the electric ray, Torpedo nobiliana . In 1886, Sir Victor Horsley began performing cortical stimulation for intraoperative mapping of focal epileptogenic lesions in the brain and, together with Clarke, developed an early stereotaxic frame . Krause, Foerster, and Penfield further used direct cortical stimulation during epilepsy surgery to develop topographic maps of human movement and the senses, codified in the Penfield homunculus .
From these early roots, surgical neuromodulation has developed into a core component of the clinician’s armamentarium in treating drug refractory epilepsy (DRE). DRE is defined as failure to attain seizure freedom despite trials of at least two appropriate and well-tolerated antiseizure medications and affects 30%–35% of children with epilepsy . Treatment options include surgical resection, disconnection, and neuromodulation. In select children, level I evidence supports surgical resection of epileptogenic tissue to improve seizure control compared with medical therapy alone . However, a number of clinical considerations may preclude the use of resective surgery to treat DRE. In cases of epileptic foci within eloquent cortex, or when no localization can be found on preoperative multimodal assessment, additional management strategies beyond resective surgery are needed. Neuromodulation has therefore arisen as a promising neurotherapeutic that may reduce seizure burden in these children.
While a number of neuromodulatory strategies for epilepsy have been proposed, the present chapter will focus on the three most effective and widely utilized techniques, namely, (1) vagus nerve stimulation (VNS), (2) deep brain stimulation (DBS), and (3) responsive neurostimulation (RNS). VNS and DBS most commonly employ open-loop (continuous) neurostimulation, while RNS distinguishes itself through a closed-loop stimulation paradigm that tailors stimulation in response to specific physiological parameters . In light of the depth, diversity, and ongoing development of these technologies, the present chapter provides a practical clinical perspective with regard to usage, advantages, limitations, and future directions as they relate to each of these promising neurotherapeutics.
Vagus nerve stimulation
The history of VNS dates back to 1938, when Bailey and Bremer discovered that electrical stimulation of the vagus nerve resulted in cortical electrographic changes in cats . In the decades that followed, multiple works evaluated the effects of VNS culminating in the 1985 work of Zabara et al. demonstrating termination of seizures in dogs in response to VNS . Such works led to the first human VNS implantation in 1988 by Penry et al. . VNS was subsequently approved by the FDA in 1997 as an adjuvant therapy for adults with focal epilepsy . It has since been adopted and approved for the pediatric population for patients as young as four years old ( Table 17.1 ). It has also been used off-label in younger children, including those aged 0-3 years . It has also been applied to various patient populations such as those with tuberous sclerosis complex (TSC) and Lennox-Gastaut syndrome (LGS).
VNS | DBS | RNS | |
---|---|---|---|
Common usage in pediatric patients | Focal (FDA approved) and generalized epilepsy (Off-label in pediatrics) in children older than four | ANT-DBS: focal and secondary generalized epilepsy (Off-label in pediatrics) CM-DBS: Class I evidence for LGS (Off-label in pediatrics) | Focal epilepsy arising from 1 to 2 foci. (Off-label in pediatrics) |
Implant location | Cervical vagus nerve electodes + subclavicular IPG | ANT or CM nucleus of thalamus electrodes + subclavicular IPG | Intracranial electrodes + cranial IPG |
Advantages | Approved in children based on high-quality pediatric data; for focal epilepsies. Data supporting use in generalized epilepsies (e.g., LGS); Seizure onset localization not necessary; Least invasive | Potential efficacy in focal/secondary generalized epilepsies (ANT) or LGS (CM); Seizure onset localization not necessary | Can be directly applied to up to 2 eloquent foci; Less overall stimulation delivered; Closed loop stimulation |
Limitations | Up to 50% of patients remain refractory to treatment | More invasive compared to VNS; multi-stage procedure | Relatively limited pediatric efficacy data; more invasive compared to VNS |
MR-conditional | ✓ | ✓ | ✓ |
The VNS device consists of two parts: the electrode with three helical contacts and the implantable pulse generator (IPG). Two contacts form a bipolar stimulating electrode, and the third serves as an anchor ( Fig. 17.1A ). The implantation technique, originally outlined by Reid in 1990, remains the current standard .

With the patient supine, neck extended, and head slightly turned right, a neck incision is marked midway between the mastoid and clavicle. The incision extends laterally from the midline to the medial border of the left sternocleidomastoid muscle (SCM). The left Vagus nerve is generally preferred as the right Vagus nerve innervates the sinoatrial node. Its stimulation has a higher likelihood of causing bradycardia and other cardiac side effects . However, in cases where left-sided VNS may not be an option (e.g., postinfection), right-sided VNS has been safely performed in children . The neck incision, measuring 3–4 cm, is made first. After dividing the platysma, the medial border of the SCM is identified and followed down to the carotid sheath. The Vagus nerve is carefully dissected for a length of 3–4 cm, taking care not to damage the vasa vasorum and perineurium sheath. Subsequently, a 5-cm chest incision is marked 5 cm below the clavicle. The chest incision is opened to create a pocket over the pectoralis muscle. The helical electrode contacts are then attached to the Vagus nerve in a specific sequence: tethering contact (lowest), positive contact (middle), and negative contact (upper). Electrode placement below the cardiac branches minimizes cardiac side effects. The electrode lead is tunneled to the chest incision and connected to the IPG. Electrode impedance is checked; a high impedance (>1700–2000 Ω) suggests poor contact. During this stage, the anesthesiologists should be alerted to possible bradycardia or asystole risks. Once impedance is verified, the battery is secured to the pectoralis fascia using nonabsorbable sutures, and the lead anchors are sutured to the SCM. The surgical sites are cleansed with vancomycin irrigation, and the incisions are closed in layers using absorbable sutures. A final impedance check ensures optimal electrode-nerve contact postoperatively .
VNS implantation carries the risk of postoperative complications, such as hematoma (1.9%), infection (2.4%), vocal cord palsy (1.4%), and lower facial weakness (0.2%). Hardware-related issues also arise in a minority of cases: lead fracture or malfunction occurs in 3.0% of patients, and spontaneous VNS activation and lead disconnection each in 0.2%. Other complications include pain and sensory problems (1.4%), aseptic reactions, cable discomfort, surgical cable breakage, oversized stimulator pockets, and battery displacement, each occurring in 0.2% of patients.
The majority of studies on VNS have focused on patients older than 12 years of age. For patients with DRE, the multicenter, blinded randomized trials E03 and E05 have shown that high-intensity stimulation with VNS can significantly improve seizure frequency compared to low-intensity stimulation . The E03 study randomized 114 patients to either 14 weeks of high-intensity or low-intensity stimulation. Patients in the high-intensity group had a mean seizure reduction of 24.5% compared to 6.1% in the low-intensity group. Furthermore, 31% of patients in the high-intensity group had >50% reduction in seizures, compared to only 14% in the low-intensity group. The E05 study subsequently randomized 196 patients to similar groups and observed outcomes at 3 months. They noted that patients in the high-intensity group had significantly higher seizure reductions compared to the low-intensity group (28% vs 15%). A 2011 metaanalysis by Englot et al. presents the efficacy data for VNS in 3321 patients across 74 clinical studies . The fraction of patients achieving >50% response ranges from 21% to 57% in these studies. This led the authors to conclude that 50% of patients achieve a 50% or greater reduction in their seizures—a response rate that remains commonly quoted to patients. Overall, VNS has demonstrated significant, albeit variable, efficacy in adolescent and adult populations.
In children, a 2021 metaanalysis by Jain et al. examined 99 articles and 3474 patients investigating VNS outcomes in patients under 18 . The primary outcome was the proportion of patients who achieved ≥50% seizure reduction at the last clinical follow-up. The authors reported that 56.4% of patients achieved the aforementioned seizure reduction target—similar to the response rates in adults. The authors further examined a subset of 1865 patients for whom seizure freedom data was available and found that 11.6% of patients achieved seizure freedom. The authors also included studies that focused exclusively on LGS and Dravet syndrome (DS) . In the subgroup of patients with LGS, 50% response was achieved by 58.2% of patients at the last follow-up. In the DS cohort, the same response was achieved by 44.7% of patients. In addition to LGS and DS, VNS has also demonstrated efficacy in patients with TSC. A metaanalysis by Hajtovic et al. included 18 studies and 63 patients who underwent VNS for DRE secondary to TSC. VNS significantly reduced seizure burden with 68% of patients achieving 50% seizure reduction, 31% achieving 90% reduction, and 40% achieving total seizure freedom. Taken together, VNS shows significant ability in improving seizure control in children with DRE, in the context of numerous pathologies and syndromes.
While the exact mechanisms of action of VNS are not fully understood, a complex network of signals between the brainstem and cortical structures—termed the vagus afferent network (VagAN) is thought to be the means of VNS treatment effect . Nearly 80% of vagal fibers carry afferent sensory input relayed to the nucleus tractus solitarius in the brainstem. From here, there are widespread projections to multiple brainstem centers, including the locus coeruleus, dorsal raphe nucleus, and the parabrachial nucleus that then project diffusely to higher cortical regions such as the thalamus, somatosensory cortex, anterior cingulate, and prefrontal cortex . Through these pathways, VNS is thought to result in increased noradrenergic signaling within limbic structures in association with treatment response . VNS also results in modulation of thalamocortical circuits . Increased thalamic activation and higher preoperative thalamocortical connectivity portends better response to therapy .
VNS is an effective palliative treatment option for children with DRE who have either failed or are not candidates for resective surgery. Metaanalyses of thousands of children and adults have demonstrated a robust benefit in select patients . However, half the patients undergoing VNS do not experience meaningful benefits despite the risk of associated complications . Complications that also include programming-related ones such as hoarseness, voice alterations, paresthesia, cough and shortness of breath . Further work is therefore needed to optimize treatment outcomes by identifying ideal programming strategies and biomarkers predictive of response .
Deep brain stimulation
Originally developed in the late 1980s to treat refractory Parkinson’s disease , DBS is also gaining traction as a treatment for certain forms of DRE. In this context, various targets such as the anterior nucleus of thalamus (ANT), centromedian (CM) nucleus of the thalamus, hippocampus, cerebelleum and nucleus accumbens have been investigated but the ANT and CM are the only targets supported by class I evidence ( Table 17.1 ) .
DBS implantation for pediatric epilepsy demands meticulous technique. Target regions such as the ANT or CM thalamus can be identified both directly via visualization on imaging, and indirectly based on the anterior commissure-posterior commissure (AC-PC) plane. The ANT can be directly visualized on 3T MRI by identifying key landmarks such as the mammillothalamic tract (MMT), external and internal medullary laminae . Novel sequences such as FGATIR can further improve visualization of the MMT and help localize the ANT . Indirect targeting of the ANT is now rarely performed. General coordinates that have historically been applied are: 12 mm superior, 5–6 mm lateral, and 0–2 mm anterior to the mid-commissural point, . Direct visualization of the CM on MRI is best achieved with sequences such as MP2RAGE, where the CM appears as a hyperintense region with its medial and posterior boundaries marked by hypointense regions corresponding to the mediodorsal and pulvinar nuclei, respectively . At our institution, we prefer the FGATIR sequence, which provides good direct visualization of the CM. To target the CM indirectly, the electrode is positioned roughly 10 mm from the midline in the posterior commissural plane . The initial step of stereotactic frame placement is commonly performed using a Leksell frame parallel to the AC-PC plane while noting to center the patient’s head in the frame. The frame’s pins should be secured firmly to the child’s head to prevent displacement but not overtightened to avoid frame distortion, and care must be taken to avoid fracture or breach of the inner table of the calvarium. Following frame placement, high-resolution CT or CTA scans inform preoperative trajectory planning, aiding in optimal burr hole placement avoiding surface vessels.
Once the patient is in the operating room, they are placed supine with a neutral spine position and their head secured. An incision is made based on the planned trajectory entry points. Burr holes are drilled perpendicularly to the skull at these locations, and lead fixation rings are subsequently attached. The dura mater is then incised and cauterized, followed by a corticotomy. Stereotactic alignment to the preplanned trajectory is then carried out, and a cannula is advanced toward the target. At our centre we prefer to perform the technique under fluoroscopic guidance. To mitigate the risk of cerebrospinal fluid (CSF) leakage and pneumocephalus, we recommend the application of gelfoam and sealant to seal the burr hole before fully inserting the cannula.
The lead is then advanced through the cannula and secured to the fixation ring. Its final position is confirmed via fluoroscopy after the cannula is removed. Leads are tunneled subgaleally toward the right posterior region. The incision is meticulously irrigated and closed in layers, and the patient is removed from the stereotactic frame. The final step is the IPG implantation. For this, the patient is positioned supine with the shoulder elevated on the right side, and an infraclavicular incision is made to create a subfascial pocket. A small incision at the leads allows for tunneling the extension leads toward the infraclavicular pocket. The extension leads are connected to the IPG. An impedance check confirms the system’s integrity. Excess lead slack is coiled below the IPG, and the surgical sites are irrigated with antibiotic solution and vancomycin powder before layered closure ( Fig. 17.1B and C ).
The majority of DBS research has focused on adult patients, which has made direct inference for pediatric populations more challenging . In adults, the stimulation of the anterior nucleus of thalamus for epilepsy (SANTE) trial provides level I evidence in support of ANT-DBS to treat partial and secondary generalized DRE. This double-blind, multicenter, randomized controlled trial examined 110 adult patients. After adjusting for prespecified covariates, a 29% greater reduction in seizures was observed with ANT-DBS compared to the control arm . In addition to the SANTE trial, several observational studies have demonstrated seizure reductions ranging from 48% to 76% following ANT-DBS, particularly for temporal-onset seizures . CM-DBS is more commonly employed in patients with electroclinical characteristics of LGS . For young adults with LGS, the DBS of Thalamic Centromedian Nucleus for Lennox-Gastaut Syndrome (ESTEL) trial provides level I evidence supporting CM-DBS in this population. Although no significant difference in patient-reported seizures was found, CM-DBS significantly reduced electrographic seizure occurrence by a median difference of 57% compared to control subjects . Two further controlled clinical studies support the potential efficacy of CM-DBS in DRE. Fisher et al. studied seven patients with DRE, of whom two were diagnosed with LGS. They observed a 30% seizure reduction with CM-DBS in comparison to sham stimulation, though this result did not attain statistical significance. Velasco et al. reported that long-term CM stimulation was most effective in patients with LGS (81% seizure reduction) when compared to patients with focal epilepsy (57% seizure reduction). In concordance with the ESTEL trial, a significant reduction in prototypical generalized spike-wave discharges was observed in patients with LGS .
While DBS has not yet been FDA-approved for children with DRE, there multiple works in pediatric literature that investigate its efficacy. Yan et al. conducted a systematic review of DBS targeting multiple brain regions for the treatment of DRE in children. Across 21 studies, eight children who had undergone ANT-DBS and 18 with CM-DBS were identified. Six children treated with ANT-DBS experienced significant seizure reductions . CM-DBS was associated with a 30%–100% seizure reduction in 17 of the 18 participants, 9 of whom had LGS . The optimal stimulation parameters utilized in these studies varied between subjects. Broadly speaking, ANT-DBS in children tends to exhibit the greatest effects with a 100–200 Hz frequency, 9–16 µs pulse width, and 1.5–8 V voltage; CM-DBS stimulation frequency has been reportedly more variable (60–130 Hz) at a 100–450 µs pulse width and voltage ranging from 6 to 8 V . At our institution, we favor continuous high frequency stimulation for both ANT- and CM-DBS to mitigate side-effects that may be evident with cyclic programming. While data in children remains lacking, the recent ADVANCE (Add-on deep brain stimulation versus continuous vagus nerve stimulation for childhood epilepsy) provides evidence for add-on DBS in children who did not demonstrate a seizure response to VNS. With the combination of DBS and VNS, a 51.9% decrease in seizure frequency was reported after 1 year, compared to a 12.3% decrease in children who continued with VNS optimization alone . The additive and synergistic effects of different neuromodulation modalities warrants further attention in forthcoming studies.
Specific considerations necessitating further study in children include the risk of procedural complications, predictors of response to ANT- or CM-DBS, and ethical concerns related to vulnerable pediatric populations. In their review, Yan et al. found only four complications, all of which were infection, in the 40 children studied . However, a paucity of long-term data precludes the assessment of additional complications that may arise secondary to growth and development, such as electrode or wire migration. Furthermore, little is known with regard to patient selection and response prediction beyond the suitability of ANT-DBS for focal-onset seizures and CM-DBS for LGS and potentially generalized-onset epilepsies, such as idiopathic generalized epilepsy . Finally, given that thalamic DBS in children remains an off-label treatment, several ethical considerations arise. These include: (1) challenges with obtaining freely informed consent or assent from children and their families for experimental therapeutics; (2) ensuring that DBS research is conducted in the best interest of the child; and (3) the challenge of basing treatment decisions on predominantly adult data . Surgeons and clinicians must carefully consider such potential ethical pitfalls when deciding to offer DBS treatment, particularly in comparison to more established neurotherapeutics in children such as VNS.
Mechanistically, ANT-DBS is thought to modulate limbic circuitry and has been shown to decouple thalamic and hippocampal activity in association with treatment response . While the mechanisms of CM-DBS are less well understood, modulation of the brainstem reticular formation and somatomotor cortices has been implicated in optimal response . Consistent with the demonstrated role of these brain regions in spike-wave discharge propagation , modulation of these specific circuits may in part explain the observed efficacy of CM-DBS in patients with LGS.
Taken together, thalamic DBS represents a promising neurotherapeutic for children with DRE who are not candidates for surgical resection. In particular, ANT-DBS is well-suited to the management of focal onset and secondary generalized seizures and CM-DBS for LGS and potentially generalized epilepsies. Although level I evidence now exists supporting thalamic DBS in adults, pediatric data remain limited. Therefore, while we remain optimistic about this emerging neurotherapeutic for DRE, more high-quality controlled clinical studies examining the safety and efficacy of these interventions in children are warranted.
Responsive neurostimulation
RNS is a neuromodulation modality approved by the FDA in 2014 for the treatment of DRE in adults ( Table 17.1 ) . While VNS and DBS systems deliver open-loop (continuous) stimulation; RNS instead distinguishes itself through the ability to provide closed-loop adaptive neurostimulation .
The RNS device is implanted cranially with two components. The system can be used either with depth electrodes or cortical strip leads and an IPG . Prior to implantation, intracranial EEG is used to identify up to two seizure foci that the device can record from and stimulate simultaneously . Although, the ongoing NAUTILUS study is also evaluating the efficacy of thalamic RNS in patients older than 12 years .
The implantation procedure begins with thorough presurgical planning to identify the seizure foci . The patient is then brought to the operating room and placed under general anesthesia. The responsive neurostimulator and leads are implanted based on the localized seizure onset zone. Similar to DBS, CT merged with preoperative MRI confirms final trajectory planning, avoiding cortical vessels, sulci, and ventricles ( Fig. 17.2 ).
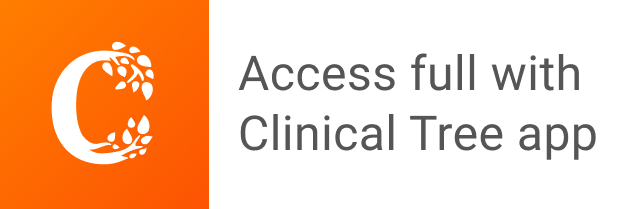