Abstract
As progenitor cells proliferate and differentiate into neurons and glia (see Chapter 5 ), these cells migrate to form the cerebral cortex in an elaborate and still not completely understood series of genetically influenced processes. Neurodevelopmental studies as well as disorders of brain development have informed our knowledge of these processes. In fact, the period of early migration overlaps with the proliferative period, and the period of late migration overlaps with later cortical organization (see Chapter 7 ). The disorders referred to as “disorders of migration” are so termed if migration is thought to be the first or principal process of development affected.
Keywords
neurogenetics, neuronal migration, lissencephaly, periventricular heterotopia, polymicrogyria, schizencephaly
Migration
As progenitor cells proliferate and differentiate into neurons and glia (see Chapter 5 ), these cells migrate to form the cerebral cortex in an elaborate and still not completely understood series of genetically influenced processes. Neurodevelopmental studies as well as disorders of brain development have informed our knowledge of these processes. In fact, the period of early migration overlaps with the proliferative period, and the period of late migration overlaps with later cortical organization (see Chapter 7 ). The disorders referred to as disorders of migration are so termed if migration is thought to be the first or principal process of development affected.
Normal Development
Neuronal migration refers to the remarkable series of events whereby millions of neurons move from their sites of origin in the ventricular and subventricular zones to the loci within the central nervous system (CNS), where they will reside for life. The peak time period for this occurrence is from the third to fifth months of gestation, although neuronal migration can be detected in certain areas of the cerebrum as early as the second month and after the fifth month (especially GABAergic interneurons; see later) ( Table 6.1 ). Regulation of the timing and direction of these many simultaneous migrations must be highly ordered, but only recently has insight been gained into these control mechanisms (see later).
Peak time period |
3–5 months |
Major events |
Cerebrum |
Radial migration: cerebral cortex (projection neurons), deep nuclei Tangential migration: cerebral cortex (interneurons) |
Cerebellum |
Radial migration: Purkinje cells, dentate nuclei |
Tangential migration: external → internal granule cells |
Radial and Tangential Migration
The major features of cell migration in the primate were defined initially, particularly by the classic studies of Sidman and Rakic, using primarily autoradiographic, electron microscopic, and Golgi techniques. Later work used immunocytochemical and retroviral methods and the study of genetically manipulated animals to elaborate earlier observations. Two basic varieties of cell migration have been delineated: radial and tangential (see Table 6.1 ). In the cerebrum , radial migration of cells from their origin in the ventricular and subventricular zones is the primary mechanism for the formation of the cortex and deep nuclear structures. Radial migration gives rise to the projection neurons of the cortex. These neurons emanate primarily from the dorsal region of the subependymal germinative zones.
Tangential migration of neurons generated in the dorsal and ventral telencephalon, detailed in Chapter 5 , results in the gamma-aminobutyric acid (GABA)–expressing interneurons of the cerebral cortex. a
a References .
These neuronal precursors migrate parallel to the surface of the cortex and proceed in one of three streams (i.e., through the subventricular zone, the intermediate zone, or the marginal zone) before terminal radial movement to arrive in the cortical plate.In the cerebellum , radial migration causes the genesis of Purkinje cells, the dentate nucleus, and other roof nuclei. Tangential migration of cells that originate in the germinal zones in the region of the rhombic lip and migrate over the surface of the cerebellum forms the well-known external granular layer . These cells then migrate radially inward to form the internal granule cell layer of the cerebellar cortex. Thus, during their journey from their point of origin in the ventricular zone, the granule cells exhibit both radial and tangential migration.
Migration to Cerebral Cortex
The basic patterns of cell migration for formation of the cerebral cortex are shown in Figs. 6.1 and 6.2 . The first and earlier mechanism is movement by translocation of the cell body (i.e., somal translocation) ( Table 6.2 ). This mode of migration probably results in the formation of the preplate (see Fig. 6.1 ). This layer of neurons is later split by the arrival of the cortical plate neurons in a superficial layer nearest the pial surface, which produces the Cajal-Retzius and related neurons of the marginal zone and a deeper layer, which becomes the subplate neurons. The preplate neurons and the subsequently formed Cajal-Retzius and subplate neurons are critical for the progression of neuronal migration (see later).


Initially, neurons migrate by translocation of the cell body (somal translocation). Later and predominantly, neurons migrate by following radial glial guides (i.e., the fibers of their clonally related radial progenitors). |
Simultaneous with this radial migration, tangential migration parallel to the surface of the cortex (followed by radial migration to the cortex) results in the placement of GABA-expressing interneurons throughout the cortex. |
Proliferative units of the ventricular zone migrate along the radial glial scaffolding to become the ontogenetic neuronal columns of cerebral cortex. |
Migration through subplate neurons and “waiting” thalamocortical and corticocortical afferents is likely important for later neuronal development (e.g., synaptogenesis). |
Early-arriving neurons take deep positions in cortex, and later-arriving neurons take superficial positions (i.e., inside-out pattern). |
The second mode of migration, leading to the formation of most of the cerebral cortex, occurs by radial migration (see Figs. 6.1 and 6.2 ). These cells are generated by the radial glial progenitors discussed earlier (see Chapter 5 ). The clonally related neuron migrates along the parental radial glial fiber, which extends to the pial surface. Initially, cells are generated in the ventricular zone and then migrate relatively rapidly and synchronously through the intermediate zone in waves to the developing cortical plate. At later stages , as shown by Rakic in studies of the monkey visual cortex, the neurons are generated especially in the subventricular zone (see Fig. 6.1 ). By labeling dividing cells with [ 3 H]thymidine at various times during development and then determining where the labeled cells appear in the cortical plate, Rakic showed that cells that migrate first take the deepest positions in the cortex, whereas those migrating later take more superficial positions. By 20 to 24 weeks of gestation, the human cerebral cortex essentially has its full complement of excitatory and projection neurons, with neurons and glia having arrived from both radial and tangential migration streams and expressing various markers reflecting their neuronal identities, as schematized in Fig. 6.3 . A substantial proportion of GABAergic interneurons migrate in the latter months of gestation, such that by approximately term, GABAergic interneurons reach their peak density in the cortex.

How do the migrating cells know how to reach where they are going? In the major process of radial migration, radial glial cells serve as the guides for the migration of young neurons from their sites of origin in the ventricular and, later, subventricular zones, across a distance that can be many times greater than the length of their leading processes, to their ultimate position in the cortical plate (see Table 6.2 and Fig. 6.4 ). a
a References .
The elaboration of the structure of the radial glial fiber system has been clarified by immunocytochemical and ultramicroscopic studies, especially by Caviness and others. Initially, the system is uniformly radial in alignment, and in the ventricular zone the fibers appear to separate columns of germinative cells, the proliferative units described by Rakic in the primate (see Chapter 5 ). The radial glial system in the developing cerebral wall forms fascicles of fibers rather than isolated fibers. With the rapid growth of the cerebral wall and particularly the intermediate zone, the fiber fascicles develop distinct curves with definite region-specific changes in trajectory ( Fig. 6.5 ). Nevertheless, the dominant feature remains the migration of apparent clonally related columns of cells among the same radial glial fascicles, again likely related to the proliferative units described earlier. As the migrating neurons approach the cortical plate, the radial glial fascicles begin to defasciculate, and radial fibers tend to penetrate the cortex more as single fibers. This occurrence develops at the junction of the upper intermediate zone and the subplate zone, an important site for neuronal heterotopia in disorders of neuronal migration. As outlined in Chapter 5 , it has long been postulated that the progeny of a single daughter cell arising from asymmetrical cell division at the ventricular zone gives rise to a column of neurons and that a columnar organization is thus established. Although this framework applies generally, there is also evidence that while some clonal populations of cells maintain regional specification, partially overlapping with other, neighboring clonal populations, other populations of neurons become widely dispersed through the cortex.

Insights into the key molecular determinants of neuronal migration have been gained in recent years ( Table 6.3 ). We present a brief discussion here of some of the best-studied molecules; further review of molecular determinants is provided later in the discussions of the molecular aspects of individual disorders of migration. Roles for molecules on preplate neurons (and the later Cajal-Retzius and subplate neurons), radial glia, and migrating neurons have been established. From preplate neurons and the Cajal-Retzius cells of the marginal zone , such extracellular matrix molecules as fibronectin, chondroitin and heparan sulfate proteoglycans are clearly crucial. The secreted glycoprotein reelin, lacking in the mutant mouse “reeler” with a neuronal migrational disorder, is an important product of the Cajal-Retzius cells. a
a References .
Platelet-activating factor acetylhydrolase, lacking in one form of human lissencephaly (see later), suggests an important role for a molecule related to platelet-activating factor, a notion further supported by in vitro studies. Neurons with GABA receptors in the preplate, Cajal-Retzius cells, and perhaps migrating neurons also now appear to be involved in migrational events.Preplate neurons (also marginal [Cajal-Retzius] zone and subplate neurons) and extracellular matrix | Fibronectin, chondroitin, and heparan sulfate |
Fukutin proteoglycans | |
GABA receptors | |
Integrins | |
Laminin | |
Reelin | |
Radial glia | erb B4 receptors Notch receptors |
BLBP | |
Migrating neurons | Neuregulin |
Astrotactin | |
Doublecortin | |
Platelet activating factor acetylhydrolase (subunit 1) | |
Filamin 1 | |
Cyclin-dependent kinase-5 (cdk-5) | |
NCAM | |
NMDA receptors | |
Calcium channels | |
GABA receptors |
Important molecular determinants of migration on radial glia include three signaling pathways involving erb B4 receptors, Notch receptors, and brain lipid-binding protein (BLBP) (see Table 6.3 ). The first of these are the surface ligands for neuregulin located on migrating neurons , which express several proteins involved in migration (see Table 6.3 ). An additional and well-characterized surface molecule of importance on migrating neurons is the surface glycoprotein astrotactin. Doublecortin is the product of a gene on the X chromosome and is involved in double cortex (band) heterotopia in female patients and lissencephaly in male patients, important human neuronal migration disorders (see later). This protein, expressed in migrating neurons at their growing end, is involved in an intracellular signaling pathway important for neuronal migration. Doublecortin is a microtubule-associated protein, which plays a role in microtubule polymerization and thereby may be involved in neuronal migration by mediating the cytoskeletal changes required for such movement, including through interactions with synaptic vesicle trafficking proteins. The gene filamin 1 (or FLNA ), which is responsible for the human neuronal migration disorder periventricular heterotopia, encodes a neuronal actin cross-linking protein that transduces ligand-receptor binding into actin reorganization, critical for the locomotion of many cell types. Involvement of neuronal calcium channels, glutamate, and N -methyl- d -aspartate (NMDA) receptors is supported by the work particularly of Rakic and colleagues but also of others. Thus selective blockage of N-type but not of L- and T-type calcium channels inhibits neuronal migration. N-type calcium channels are involved primarily in the release of neurotransmitters. That the neurotransmitter involved is glutamate, which acts on the NMDA receptor, is suggested by the demonstration that blockers of NMDA receptors, but not of non-NMDA receptors, inhibit neuronal migration ( Fig. 6.6 ). Axonal release of glutamate may be the source of the glutamate that acts on the migrating neuron.

Radial glial cells have additional functions aside from guidance of neuronal migration (see Chapter 5 ). Thus the initial role of these cells is as neuronal progenitors. The generation of neurons is followed by their role as radial glial guides. Still later, these cells give rise to astrocytes and oligodendroglia. Finally, the cellular progeny of the radial glial cell serves as a source of neural stem cells in the subventricular zone of the mature brain.
Disorders
Disorders of neuronal migration usually cause overt disturbances of neurological function, with clinical deficits often apparent from the first days of life. Seizures are most often the dominant early neurological sign with the more severe migrational disturbances. The advent of magnetic resonance imaging (MRI) markedly increased the ability to identify these disorders in vivo, demonstrating the relatively high prevalence of these disorders and showing their broad clinical expression (see later discussion). The major disorders are listed in Table 6.4 in order of decreasing severity. The disorders included here are considered migrational disorders conventionally, but as indicated, some have been found through molecular genetics to be due to dysfunction that begins during proliferation and continues to exact its major effects on migration as well as subsequently on cortical organization.
Order of decreasing severity |
Schizencephaly |
Agyria-pachygyria spectrum (e.g., lissencephaly) |
Polymicrogyria |
Heterotopia—periventricular, subcortical |
Gyral Abnormality in Migrational Disorders
The hallmark of the migrational disorders is an aberration of gyral development. Formation of the many secondary and tertiary gyri of the human brain occurs after neuronal migration has ceased ( Fig. 6.7 ). The fastest increase in number of the major gyri occurs between 26 and 28 weeks of gestation. Further elaboration of these gyri continues during the third trimester and shortly after birth. The stimulus for gyral formation appears to be the remarkable increase in surface area of cerebral cortex that occurs during this period, particularly the difference in increase in surface area of the outer versus the inner cortical layers. In the normal cortex, the surface area of the outer cortical layers is greater than that of the inner layers, and this discrepancy leads to compressive stresses that may lead to gyral formation. These relative increases in cortical surface area require the complement of neurons provided by migrational events. In lissencephaly, in which all cortical layers fail to receive their full complement of neurons, no gyri develop. In polymicrogyria, the surface area of outer cortical regions is much greater than that of inner cortical regions, and the result is an excess of gyri. In addition, gyral development may be stimulated by the forces produced by growth of cerebral white matter axons originating in the cortex, the concept of tension-based morphogenesis .

Corpus Callosum Defect in Migrational Disorders
In addition to gyral abnormality, a common feature of migrational disorders is hypoplasia or agenesis of the corpus callosum; occasionally, absence of the septum pellucidum also accompanies these disorders. Development of the corpus callosum (the major interhemispheric commissure) and of the septum is associated temporally and causally with migrational events in the cerebrum (see Chapter 2 ). Thus the timing of these aspects of midline prosencephalic development is almost coincidental with the major neuronal migrational events for the formation of cerebral cortex. Moreover, normal elaboration of corticocortical callosal fibers requires normal progression of neuronal migration to cerebral cortex. The frequent concurrence of hypoplasia or agenesis of the callosum with the migrational disorders discussed is therefore understandable. The preponderance of cortical abnormality at times dominates to the point that the callosal defect is not initially noted; in some settings, however, the callosal defect is a key feature of the abnormality—for example, with ARX – and TUBA1A -associated lissencephaly, discussed later.
Schizencephaly
Anatomical Abnormality.
Schizencephaly is the most severe yet restricted of the cortical malformations (see Table 6.4 ). A complete agenesis of a portion of the germinative zones and thereby the cerebral wall is believed to exist, leaving seams or clefts. The pial-ependymal seam is characteristic ( Fig. 6.8 ). In the walls of the clefts, the cortical plate exhibits the hallmarks of migrational disturbance (e.g., a thick, microgyric cortex and large neuronal heterotopia). In bilateral lesions, schizencephaly in one hemisphere may be accompanied by polymicrogyria or focal cortical dysplasia (FCD) in the other. The lips of the clefts may become widely separated, and dilation of the lateral ventricles may occur. Hydrocephalus often complicates such open-lipped lesions, especially when they are bilateral (see later). Such bilateral open-lipped lesions may be referred to incorrectly as hydranencephaly, a later destructive lesion of the cerebral hemispheres; when they are unilateral, they may be referred to incorrectly as porencephaly, a destructive lesion of one hemisphere. Indeed, it is now clear that unilateral and bilateral schizencephalies can be familial and probably account for previous reports of “familial porencephaly.” Gray matter (often polymicrogyric), lining the lesion and demonstrable on brain imaging, especially MRI, is the key finding indicative of schizencephaly.

The advent of MRI greatly expanded the understanding of anatomical and clinical aspects of schizencephaly (see Table 6.5 ). Indeed, several previous notions that were based almost exclusively on the study of autopsy cases (i.e., that schizencephaly is rare, bilateral, and associated invariably with severe neurological deficits) were shown to be incorrect. Thus, in two large series, 67 cases were collected, and schizencephaly was unilateral in 63% (see Table 6.5 ). The clefts tended to be in the regions of the rolandic and sylvian fissures and involved predominantly frontal areas. Subsequent series confirmed these observations.
FEATURE | PERCENTAGE |
---|---|
Anatomical | |
Unilateral | 63 |
Bilateral | 37 |
Closed clefts | 42 |
Open clefts | 58 |
Frontal | 44 |
Frontoparietal | 30 |
Parietal, temporal, or occipital | 26 |
Associated septo-optic dysplasia | 39 |
Anatomical-clinical correlates | |
Cognitive disturbances (prominent) | |
Bilateral | 100 |
Unilateral | 24 |
Motor disturbances | |
Bilateral | 86 |
Unilateral | 77 |
Frontal | 84 |
Not frontal | 29 |
Open lip | 94 |
Closed lip | 22 |
Seizure disorder | |
Bilateral | 72 |
Unilateral | 60 |
Hydrocephalus | |
Open lip | 52 |
Closed lip | 0 |
Timing and Clinical Aspects.
Onset of the developmental disturbance that leads to schizencephaly is considered to be no later than the beginning of migrational events in the cerebrum in the third month of gestation. The possibility that destructive lesions operate in the third or fourth month of gestation, perhaps injuring both the germinative zones and migrating neurons on radial glial fibers, is raised by multiple reports. However, the demonstration, at least in certain rare familial cases, of a defect in the homeobox gene EMX2 supports the notion that schizencephaly most often is a disorder of the developmental program. This gene, specifically expressed in neuroblasts of the ventricular zone, is involved in the structural patterning of the developing forebrain, including neuronal migration. Nevertheless, a multifactorial origin of schizencephaly is supported by the association with fetal cytomegalovirus (CMV) infection (which can cause both dysgenetic and destructive effects) and systemic abnormalities secondary to vascular disruption. Candidate gene sequencing for the genes LHX2 , HESX1 , and SOX2 —initially thought to be associated with schizencephaly and its related condition septo-optic dysplasia—revealed that these were not common causes of the malformation. To date, genetic explanations for schizencephaly remain few; one prevailing theory is that the disorder results from a disruption of vascular development during critical times of cerebral development. More recently, however, recessive mutations in the “microcephaly” gene WDR62 have been shown to result not only in microcephaly but also in schizencephaly in some cases, implicating the processes underlying proliferation in the genesis of schizencephaly. Another genetic cause that has emerged recently is mutation of the gene COL4A1 , associated with schizencephaly plus a number of other features, including CNS findings (e.g., intracranial calcification, focal cortical dysgenesis, pontocerebellar hypoplasia) and extracerebral findings (e.g., hemolytic anemia).
The clinical features of schizencephaly have begun to be clarified by the study of patients identified by MRI (see Table 6.5 ). The lesion may be suspected because of the appearance of a focal ventricular dilation on ultrasonography or CT or occasionally because of visualization of a gray matter–lined cleft on CT ( Fig. 6.9A ). However, far more sensitive for identification of schizencephaly is MRI ( Fig. 6.9B ). The salient feature is the lining of the cleft by cerebral cortex, often thickened by pachygyric or polymicrogyric cortex with heterotopia. The clinical spectrum is clearly broader than was previously expected. Severity relates to the extent and distribution of cerebral involvement (see Table 6.5 ). In two series, prominent cognitive disturbances were present with all bilateral lesions but only 24% of unilateral lesions. (We have seen one patient who had bilateral clefts and average intelligence.) Motor disturbances are nearly invariable with frontal and open-lipped lesions (see Table 6.5 ). Seizures may begin as late as adult life in patients with schizencephaly. Hydrocephalus complicates approximately 50% of open-lipped lesions (see Table 6.5 ), although the mechanism for the impaired cerebrospinal fluid dynamics is unclear. Agenesis of the septum pellucidum occurs in 70% of cases, and accompanying septo-optic dysplasia occurs in 10% to 25% (see Chapter 1 ). Agenesis or hypoplasia of the corpus callosum occurs in 30% of cases of schizencephaly.

Lissencephaly and the Agyria-Pachygyria Spectrum
Anatomical Abnormality.
In lissencephaly (i.e., “smooth brain”), the brain has few or no gyri. a
a References .
Two basic anatomical types of lissencephaly can be distinguished ( Tables 6.6 and 6.7 ). In type I lissencephaly , the cerebral wall is similar to that of an approximately 12-week-old fetus ( Fig. 6.10A and B ). The layers consist, from the pial surface, of an outermost relatively cell-poor marginal layer, a diffuse cellular layer containing primarily pyramidal and other neurons characteristic of lower layers of cortex, a zone of heterotopic neurons in columns, and an innermost band of white matter. The pathological features indicate that the diffuse cellular layer contains neurons that were destined to constitute the deep layers of cortex but were never displaced by subsequent migrations, the neurons of which constitute the heterotopic layer. In type II lissencephaly the appearance is quite different (see Fig. 6.10C ). The cortex is represented by clusters and circular arrays of neurons, with no recognizable organization or lamination, separated by glial and vascular septa. Large heterotopic collections of neurons are prominent. Notable, however, are the protrusions over the cortical surface where neurons have migrated through the pia. These protrusions cause the cortical surface to be irregular or “pebbly” in appearance.DISORDERS | GENE LOCUS | PROTEIN | FUNCTION |
---|---|---|---|
Isolated lissencephaly (LIS1) | 17p13.3 | PAFAH1B1 | Cytoskeleton (microtubules/dynein) |
Miller-Dieker syndrome (LIS1) | 17p13.3 | PAFAH1B1 | Cytoskeleton (microtubules/dynein) |
14-3-3ε | |||
Isolated lissencephaly (XLIS) | Xq22.3 | Doublecortin | Cytoskeleton (microtubules) |
XLAG | Xp22 | ARX | Homeobox |
LCHb | 7q22 | Reelin | Neuron/radial glial cell interactions |
DISORDER | GENE LOCUS | PROTEIN | FUNCTION |
---|---|---|---|
Fukuyama congenital muscular dystrophy | 9q31-32 | Fukutin | Glycosylation |
Walker-Warburg syndrome | 9q34.1 | POMT1 | Glycosylation |
Muscle-eye-brain disease | 1p34 | POMGnT1 | Glycosylation |

In pachygyria , the features are similar to those described for lissencephaly but are less marked. a
a References .
The gyri are relatively few, are unusually broad, and are associated with an abnormally thick cortical plate ( Fig. 6.11 , parasagittal region). The microscopic features are similar to those of lissencephaly, although the abnormalities are less marked ( Fig. 6.12 ). Pachygyria should be considered on a continuum with lissencephaly , because, depending on the nature of the genetic disturbance, lissencephaly syndromes may be characterized principally by pachygyria rather than by lissencephaly (see later).

Timing.
The onset of lissencephaly-pachygyria is considered to be no later than the third and fourth months of gestation. This conclusion is based on the anatomical features of the lesions as well as cases in which putative teratogenic exposures could be documented.
Clinical Aspects: Type I Lissencephaly.
The clinical aspects of lissencephaly-pachygyria are considered best in terms of those disorders characterized by type I and type II disease (see Tables 6.6 and 6.7 ). Several genetic disorders are recognized in association with type I lissencephaly. Approximately 60% of these cases are caused by defects of the chromosome 17p13.3 gene ( LIS1 ), and approximately half of the remaining cases are caused by defects of the Xq22.3 gene XLIS or DCX (see Table 6.6 ). (The XLIS cases account for nearly all the male infants in the non- LIS1 group.) Both lissencephaly with cerebellar hypoplasia b (reelin deficiency) and X-linked lissencephaly with abnormal genitalia (XLAG), associated with mutations in the gene ARX , are much less common. The radiographic features described with some of the newly reported “tubulinopathies” (e.g., caused by mutation in TUBA1A ) reveal lissencephaly and pachygyria in some of these cases, often with associated abnormalities of the basal ganglia, corpus callosum, and posterior fossa.
The clinical features of the major form of type I lissencephaly ( LIS1 , isolated lissencephaly related to the chromosome 17p13.3 locus) are summarized in Table 6.8 . a
a References .
Despite the major brain anomaly, microcephaly usually is not present at birth but characteristically develops in the first year. The craniofacial appearance is generally unremarkable except for bitemporal hollowing of the skull and a small jaw. Marked hypotonia and paucity of movement are characteristic. Spasticity does not develop until later in the first year or even after that. Neonatal seizures can occur, but characteristically seizures develop in the first 6 months as infantile spasms or as akinetic-myoclonic seizures with grossly disordered findings on the electroencephalogram (EEG) (Lennox-Gastaut syndrome). The EEG is always abnormal; particularly characteristic is high-amplitude, fast activity, occurring in approximately 75% of patients with type I lissencephaly. Bursts of sharp and slow-wave complexes, interspersed with periods of voltage depression, are also common features of the EEG. Infants with primarily pachygyria rather than lissencephaly have less severe clinical deficits, including occasionally only mild subsequent impairment of intellect. In general, however, neurological outcome in LIS1 isolated lissencephaly is characterized ultimately by pronounced intellectual disability, spastic quadriparesis, and seizures.Normal head size at birth → microcephaly in the first year |
Hypotonia → hypertonia later in infancy |
Paucity of movement, feeding disturbance |
Seizures (common evolution to infantile spasms or Lennox-Gastaut syndrome in early infancy) |
Electroencephalogram severely disordered with high amplitude and rapid frequency |
The clinical features of the second major form of type I lissencephaly related to the chromosome 17p13.3 locus, the Miller-Dieker syndrome , are different from those of isolated lissencephaly because of the additional presence of craniofacial abnormalities. a
a References .
Thus, in addition to the bitemporal hollowing and small jaw observed with isolated lissencephaly, patients have a characteristic facial appearance with a short nose with upturned nares, a long and protuberant upper lip with a thin vermilion border, and a relatively flattened midface ( Fig. 6.13 ). Additional characteristic features of Miller-Dieker syndrome include cardiac malformations (20% to 25%), genital anomalies in male infants (70%), a sacral dimple (70%), deep palmar creases (65% to 70%), and clinodactyly (40% to 45%). Neurological features are similar to those described for isolated lissencephaly, although generally the disturbances are even more marked, consistent with the uniformly severe degree of the lissencephaly.
The clinical aspects of the major X-linked form of type I lissencephaly (i.e., those related to a defect in the DCX [or XLIS ] gene), are similar to those described for isolated lissencephaly caused by LIS1 . The clinical features, of course, occur in hemizygous male infants, whereas heterozygous female infants exhibit subcortical band heterotopia (see later).
The clinical aspects of the rarer form of X-linked lissencephaly (i.e., XLAG , associated with mutations in ARX ) are somewhat distinctive. Particularly characteristic features include severe neonatal seizures (onset in >50% in the first hour of life and in utero in 20%), hypothermia, severe diarrhea, and ambiguous genitalia (micropenis, cryptorchidism). The epileptic syndrome is especially severe and relates to the particular involvement of GABAergic interneurons (see later). The lissencephaly, typically posterior-predominant as with LIS1 , is accompanied also by complete agenesis of the corpus callosum. The full syndrome occurs in hemizygous male infants, although less severe phenotypes occur as a function of the severity of the genetic abnormality. Heterozygous female infants often exhibit agenesis of the corpus callosum and epilepsy.
The clinical aspects of lissencephaly with cerebellar hypoplasia b , related to a defect in RELN , which encodes reelin, are difficult to define decisively because of the paucity of careful clinical descriptions. Perhaps most notable is the presence of microcephaly at birth , rather than the postnatal development of microcephaly, as in other varieties of type I lissencephaly. The term lissencephaly with cerebellar hypoplasia b is used to distinguish these cases from lissencephaly with cerebellar hypoplasia a. The latter are examples of type I lissencephaly secondary to either LIS1 or DCX gene defects (see earlier), which may be accompanied by prominent hypoplasia of the cerebellar vermis and mild hypoplasia of the cerebellar hemispheres. By contrast, the reelin-related cases exhibit severe hypoplasia of the entire cerebellum, which also lacks folia.
The radiological features of type I lissencephaly may include a distinctive appearance on CT ( Fig. 6.14 ). However, MRI provides superior definition of the parenchymal lesion ( Figs. 6.15 and 6.16 ), particularly the appearance of a “cell-sparse zone” in the superficial aspect of the cortex. Indeed, insight into the likely genetic lesion can be gained by evaluating the degree of lissencephaly and pachygyria, any anteroposterior gradient, agenesis of the corpus callosum, and the degree of cerebellar hypoplasia (see Fig. 6.16 ). Thus, among LIS1 cases, the cortex is very thick, posterior more than anterior involvement is apparent, and Miller-Dieker cases have even more severe lissencephaly than do isolated cases. DCX cases have anterior more than posterior involvement. ARX cases have only a moderately thick cortex, posterior predominance of the abnormally thick cortex, and prominent agenesis of the corpus callosum. Reelin cases have a moderately thick, pachygyric cortex with striking cerebellar hypoplasia. A uniform accompanying feature in all genetic types is colpocephaly (i.e., dilation of the trigone, occipital horns, and temporal horns of the lateral ventricles). The ventricular dilation of colpocephaly occurs in the trigone and occipital horns because of underdevelopment of the corpus callosum and calcarine sulci and in the temporal horns because of failure of inversion of the hippocampus.



Etiology/Genetics: Type 1 Lissencephaly.
The major genetic varieties of type I lissencephaly appear to involve three different mechanisms of migrational failure (see Table 6.6 ). The LIS1 cases, both isolated lissencephaly and Miller-Dieker syndrome, and the DCX (XLIS) cases have a defect in the pace of migration. The tubulinopathies likewise result from microtubule dysfunction and resultant aberrant migration. ARX mutations appear to involve a defect in tangential more than radial migration. The RELN cases appear to be related to a defect in migrating neuron and radial glial interactions.
Isolated lissencephaly (LIS1) secondary to a deficiency in PAFAH1B1 (LIS1) involves a disturbance in migration because of abnormal function of the cytoskeleton. a
a References .
This protein is the noncatalytic alpha subunit of the isoform Ib of the platelet-activating factor acetylhydrolase. This LIS1 -encoded protein interacts with microtubules and cytoplasmic dynein motors, crucial for both somal translocation and cell motility.Miller-Dieker syndrome , of course, shares the PAFAH defect, but because the disorder relates to a deletion, other genes are disturbed. b
b References .
The protein 14-3-3ε appears to be the key additional defect in this more severe lissencephaly syndrome. Deficiency of 14-3-3ε results in mislocalization of the LIS1 protein; this accentuates the dynein motor disturbance and thereby neuronal migration.Isolated lissencephaly, secondary to the X-linked DCX (XLIS) gene and deficiency of doublecortin , appears also to be mediated at the level of the cytoskeleton. Doublecortin is a microtubule-associated protein that binds to tubulin and is necessary for microtubule polymerization. Thus, as with the two LIS1 disorders, a defect in the cytoskeleton and thereby in cell motility results.
XLAG involves a second mechanism of migrational disturbance. The gene involved, ARX , is a homeobox gene that encodes a protein critical for tangential migration. The result of the mutation is a marked deficiency of GABAergic interneurons because, as noted earlier, tangential rather than radial migration is the principal mechanism for the movement of neurons destined to be interneurons from the ventricular zone to the cortex. The severe deficiency of GABAergic neurons likely underlies the striking clinical feature of prenatal and early neonatal seizures and intractable infantile seizures (see earlier discussion of clinical aspects).
Lissencephaly with cerebellar hypoplasia b , related to the RELN gene, involves a third mechanism of disturbed migration. RELN encodes reelin, a glycoprotein secreted by horizontally oriented Cajal-Retzius cells in the preplate and marginal zones; it is crucial for signaling to migrating neurons on radial glial cells. Reelin appears to function as a stop signal and in neuronal and radial glial cell interactions. The result of reelin deficiency is a cortex that is abnormally cellular in its most superficial zone and is inverted (i.e., early migrating pyramidal cells are the uppermost layer). Preplate-like cells accumulate in the marginal zone, a finding suggesting that the preplate has not been split by the migrating cortical neurons. Related phenomena occur in cerebellum to cause the severe cerebellar hypoplasia.
The tubulinopathy family of genes frequently plays a role in lissencephaly or pachygyria, sometimes accompanied by other MRI features, as noted earlier. The genes thus far implicated include TUBA1A , TUBB2B , TUBB3 , TUBB5 , and TUBA8 , typically with de novo mutations.
Other causes of type I lissencephaly, albeit rare, are worthy of note. A role for vascular insult during the third to fourth months of gestation has been suggested. Moreover, lissencephaly/pachygyria has been documented with fetal CMV infection (see Chapter 34 ) and a variety of inborn errors of metabolism (e.g., pyruvate dehydrogenase deficiency, Zellweger syndrome, glutaric acidemia [type 2, nonketotic hyperglycinemia]; see Chapter 27 , Chapter 28 , Chapter 29 ). Rare autosomal recessive forms with marked neonatal microcephaly and lissencephaly, the most severe form of microcephaly with simplified gyri discussed among proliferative disorders, have been reported (see Chapter 5 ).
Recurrence risks for type 1 lissencephaly depend on the genetic type. Thus, LIS1 isolated lissencephaly, which is caused by de novo mutations, has a recurrence risk of approximately 1% to 10% (because of the theoretical risk of germline mosaicism in either parent). Miller-Dieker syndrome is related to de novo deletions in approximately 80% (recurrence risk of ≈1%); in 20%, it is related to inheritance of a deletion from a parent carrying a balanced chromosomal rearrangement. In the latter case, recurrence risk is higher and depends on the nature of the rearrangement. With XLIS lissencephaly, the mother may be a carrier even if the brain MRI does not show subcortical band heterotopia. Even if the mother is not a carrier, the risk of harboring germline mosaicism places a recurrence risk at about 1% to 10%. With XLAG, the carrier status of the mother should be evaluated because the phenotype in girls and women can be very mild. Thus far, the few families reported with reelin deficiency lissencephaly have exhibited autosomal recessive inheritance. The tubulinopathies are for the most part due to de novo mutations in a growing list of genes.
In cases presenting with classic patterns associated with the genes already noted, if a first attempt at genetic testing does not reveal a genetic etiology, one must consider the possibility of a mosaic mutation presenting with mutations that may be difficult to detect with conventional sequencing strategies but that may be detectable as clinical testing moves to the realm of next-generation sequencing.
Clinical Aspects: Type II Lissencephaly.
The three disorders consistently associated with type II lissencephaly are Fukuyama congenital muscular dystrophy, Walker-Warburg syndrome, and muscle-eye-brain disease (see Table 6.7 ). As noted earlier, these disorders are characterized by lissencephaly in which protrusions of neurons are found over the surface of the brain and thereby render a bumpy or pebbly configuration to the cortical surface. In type II lissencephaly, the ectopic clusters of neurons within the cortex, separated by fibroglial vascular tissue that extends radially from the cerebral white matter, result in a typical MRI appearance, termed cobblestone lissencephaly (see later). The migrational defect is unique and is similar for the three disorders (see later). In addition to type II lissencephaly, these disorders also share congenital muscular dystrophy as a prominent clinical feature. Fukuyama congenital muscular dystrophy and muscle-eye-brain disease are discussed most appropriately with diseases of muscle (see Chapter 33 ). The clinical aspects of Walker-Warburg syndrome are described here.
The major clinical features of Walker-Warburg syndrome in many respects are different from those for the type I lissencephalic disorders ( Table 6.9 ). Macrocephaly (84%), either apparent at birth (58%) or developing postnatally (26%), retinal malformations (100%), congenital muscular dystrophy (100%), cerebellar malformation (100%), and type II lissencephaly (100%) are characteristic. Type II lissencephaly and the retinal, cerebellar, and muscular abnormalities are necessary for the diagnosis. The neurological features (e.g., severe seizure disorders and intellectual disability) are similar to those identified for type I lissencephaly. However, the severe muscle disease, accompanied by elevated serum creatine kinase, accentuates the marked hypotonia and weakness observed with lissencephaly. Death in the first year is common.
Macrocephaly | 84% |
Present at birth | 58% |
Develops postnatally | 26% |
Type II lissencephaly | 100% a |
Cerebellar malformation | 100% a |
Ventricular dilation/hydrocephalus | 95% |
Retinal malformation | 100% a |
Anterior chamber abnormality | 76% |
Congenital muscular dystrophy | 100% a |
The radiological features are similar to those for type I lissencephaly in terms of the agyric brain and the concurrence of agenesis or hypoplasia of the corpus callosum or septum pellucidum. However, because of the different cerebrocortical microscopic pathological features (see earlier discussion), a slightly uneven cortex is apparent. Moreover, as noted earlier, irregular projections of the underlying white matter into the cortex lead to the term cobblestone lissencephaly ( Fig. 6.17 ). The latter appearance is more clearly apparent after the neonatal period. The most characteristic distinguishing features of Walker-Warburg syndrome include the presence of cerebellar malformation and, invariably, vermian agenesis or hypoplasia as well as complete Dandy-Walker malformation (50%) and posterior encephalocele (25% to 35%) ( Fig. 6.18 ; see also Fig. 6.17 ). The ventricular dilation (95%) can be distinguished from the colpocephaly of type I lissencephaly by involvement of the third and fourth ventricles and by the presence of the macrocephaly of hydrocephalus. Some of these distinguishing features are recalled by the eponymic designation for this syndrome: HARD ± E, h ydrocephalus, a gyria, r etinal d ysplasia, e ncephalocele. We suggest an alternative, CHARM ± E, to add c erebellar and m uscle.

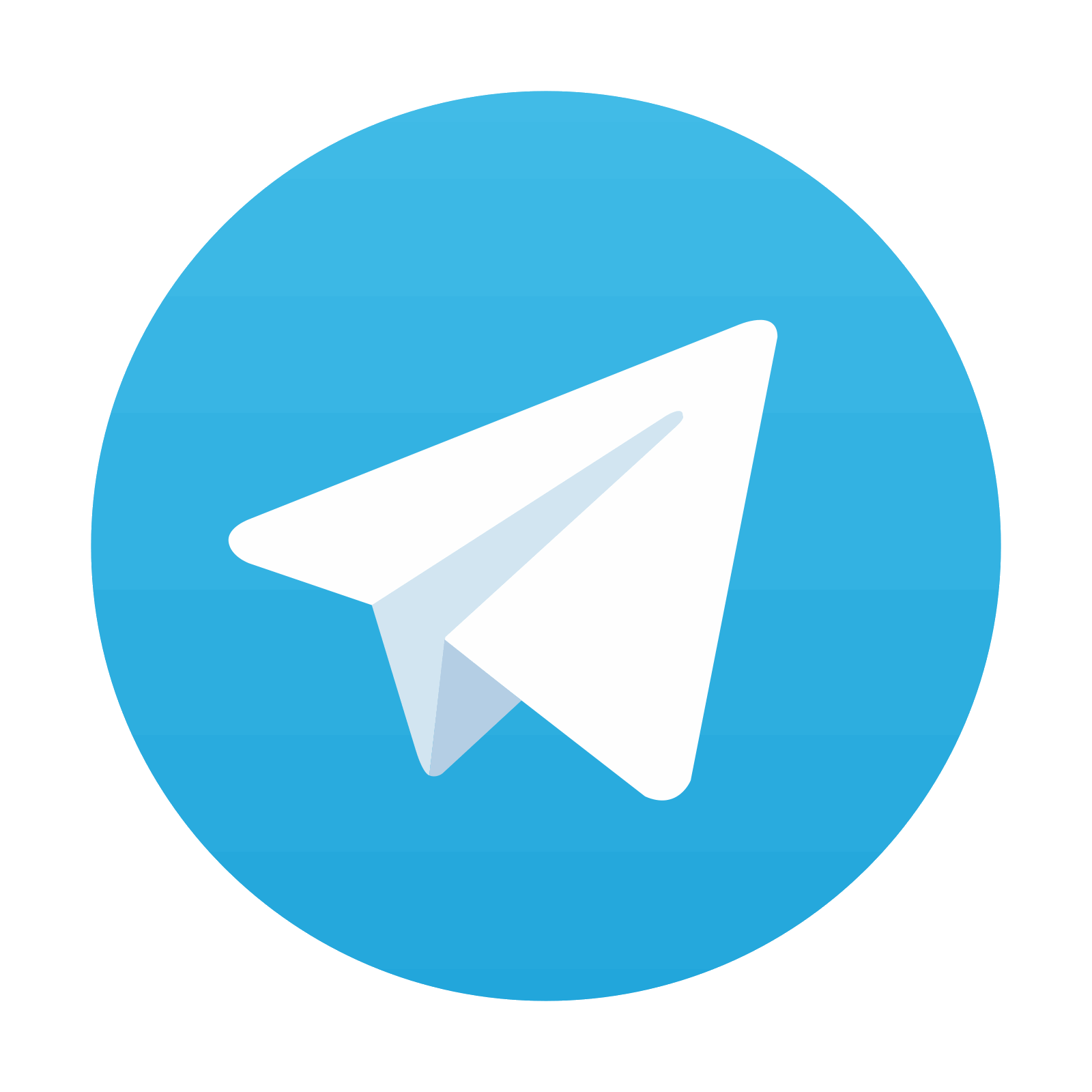
Stay updated, free articles. Join our Telegram channel
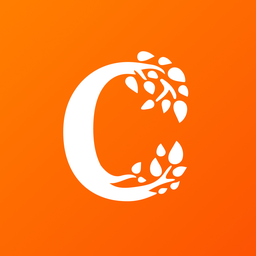
Full access? Get Clinical Tree
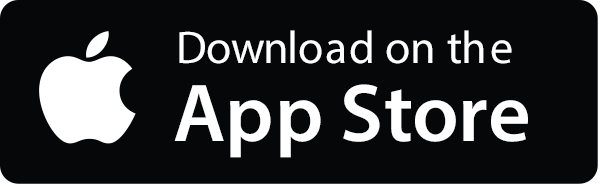
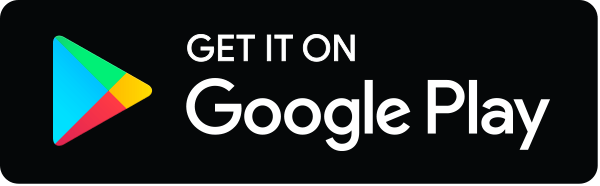
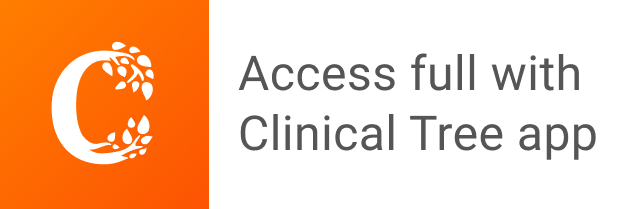