Neuronal Networks, Epilepsy, and Other Brain Dysfunctions
John G. R. Jefferys
Introduction
The dynamics of highly interconnected networks of neurones are fundamental to both normal and pathological functioning of the brain.(1, 2) Epilepsy is perhaps the most dramatic example of a dysfunctional neuronal network,(3) characterized by intense and highly synchronous neuronal activity, but more subtle dysfunction is associated with other conditions, such as schizophrenia.(4)
This chapter will largely focus on the hippocampus, and to a lesser degree on the neocortex. The hippocampal formation is implicated in several important psychiatric and neurological problems. The hippocampus and amygdala are often the site of epileptic foci, which can lead to problems in learning and memory, emotion, anxiety, and other problems. This kind of epilepsy is variously known as temporal lobe epilepsy, complex partial seizures, or limbic epilepsy. The hippocampus and associated limbic areas have been linked both to affective disorders and to psychoses. This chapter will consider the cellular organization of the hippocampus and then outline aspects of the emergent properties of neuronal networks in the hippocampus and speculative role in psychiatric disorders. Cellular and network mechanisms of focal epilepsy, and learning impairments associated with limbic epilepsy will be reviewed.
Hippocampal organization
Anatomy
The hippocampus resembles the neocortex in containing a majority of excitatory neurones, the pyramidal cells, and granule cells, which use glutamate as their neurotransmitter (E in Fig. 2.3.9.1).(1, 2) Most of the remaining 10-20 per cent of neurones in the hippocampus are inhibitory, and use γ-aminobutyric acid (GABA) as their neurotransmitter (I in Fig. 2.3.9.1). The inhibitory neurones fall into several distinct subtypes according to where their axons go (and hence which cells they inhibit), where their cell bodies are, the shapes of their dendrites, whether they contain more than one transmitter, and whether they contain particular calcium-binding proteins. This chapter will ignore most of the diversity of interneurones.(5)
There are many more excitatory pyramidal cells (E and triangles) than inhibitory interneurones (I and circles). As with most neurones, they receive inputs onto their dendrites and somata (the latter contain the nucleus and are represented by a triangle or circle). The level of simplification is clear from the observation that each pyramidal cell receives tens of thousands of synapses. Axons from other regions, known as afferents, make excitatory synapses (e) with both pyramidal cells and interneurones. Most of the interneurones make inhibitory synapses (i) onto pyramidal cells. The inhibition of the pyramidal cell is called ‘feed-forward’ (f.f.) when the interneurones were excited by afferent axons and ‘feed-back’ (f.b.) when they were excited by pyramidal cells. Interneurones also inhibit each other forming a mutually inhibitory network (m.i.); this network is important in some kinds of physiological network oscillation (see text). Finally, pyramidal cells make excitatory synapses onto each other (r.e.), which can lead to epileptic discharges if not held in check by inhibitory mechanisms.
Evoked responses
Each hippocampal (or neocortical) area receives ‘afferent’ synaptic inputs from other areas. Most afferents are excitatory; stimulating them provides a convenient tool to study the operation of the neuronal circuits involved. The responses evoked in hippocampal neurones typically start with an excitatory postsynaptic potential. If the excitatory postsynaptic potential is strong enough, it will result in an action potential triggered at a low-threshold zone near the cell body, probably a short distance down the axon. The excitatory postsynaptic potential is followed by a fast and a slow inhibitory postsynaptic potential. Both the fast inhibitory and excitatory postsynaptic potentials are due to ligand-gated channels where the transmitter receptor is part of the same molecular structure as the ion channel. In the case of inhibition this is the GABAA receptor, which allows chloride ions to pass. In the case of excitation it is a variety of glutamate receptors, which are permeable to sodium, potassium, and in some cases calcium ions, and which are further subdivided into α-amino-3-hydroxy-5-methyl-4-isoxazolepropionic acid (AMPA)/kainic acid, N-methyl-D-aspartate (NMDA) and other classes. The slow inhibitory postsynaptic potential is due to GABAB receptors, which are G protein coupled and use second messengers to open separate potassium channels. Many other kinds of G-protein couple receptors exist, and often are involved in modulating neuronal excitability or synaptic function.
Inhibitory neurones can be triggered both by activity in the principal cells (pyramidal or granule), resulting in recurrent or feedback inhibition, and directly by the incoming afferents, resulting in feed-forward inhibition. Experimentally, the synchrony of the stimulation of the afferent input imposes synchrony on the response with the useful consequence that the extracellular currents generated by the activity of individual pyramidal or granule cells can summate (because the cells are located in tight layers) and produce large ‘field potentials’ comprising a population excitatory postsynaptic potential, followed by a population spike.
Field potentials evoked by local stimulation are over in 10 to 20 ms and the slowest intracellular components end within a few 100 ms to 1 s. However, stimulation can have much more prolonged effects. The best known of these is long-term potentiation, in which a brief train of stimuli can result in an increase, lasting hours or days, in the response to a fixed test stimulus. The modest conditioning event and the enduring consequence make long-term potentiation an attractive model of learning and memory, although the evidence that it really is the direct cellular substrate for learning remains circumstantial.(6) It is perhaps more likely that long-term potentiation provides an artificial experimental tool that depends on cellular and molecular mechanisms that may also be involved in learning and/or other plastic changes in synaptic strength.
Local circuits
Hippocampal neurones are not just arranged as a simple synaptic relay where afferents excite target cells to produce an output depending on the size of the input and the state of inhibition at that time. Instead there exists a complex synaptic network, or local circuit, interlinking neurones of all kinds (Fig. 2.3.9.1 gives a very much simplified illustration of some of the salient features of hippocampal local circuits). This chapter considers two kinds of emergent network activity that arise from this organization: focal epilepsy and gamma rhythms.
Experimental approaches
Unravelling the cellular and network mechanisms of emergent network phenomena depends on a combination of electrophysiology, pharmacology, anatomy, and realistic computer simulations.(2,7) Two practical issues may need a brief introduction.
Brain slices have played a pivotal role in developing theories on the operation of neuronal networks. Slices about 0.4 mm thick are cut from the brains of deeply anaesthetized or recently killed experimental animals, or sometimes from humans undergoing
neurosurgery. Brain slices can survive many hours in vitro in an artificial cerebrospinal fluid, usually equilibrated with 95 per cent oxygen and 5 per cent carbon dioxide (with bicarbonate providing a pH buffer). If the slices are prepared under sterile conditions, they can survive for weeks as ‘organotypic slice cultures’. In both cases the visualization of the anatomy of the living slice helps locate electrodes, the mechanical stability greatly simplifies recordings from inside neurones, and the lack of a blood-brain barrier facilitates drug applications and changes in ion concentrations. Brain slices have proved immensely popular and successful, but it is important to remember that they are only one tool in the armoury needed to study brain function, and that ultimately results from them must be put in the context of the whole organism.
neurosurgery. Brain slices can survive many hours in vitro in an artificial cerebrospinal fluid, usually equilibrated with 95 per cent oxygen and 5 per cent carbon dioxide (with bicarbonate providing a pH buffer). If the slices are prepared under sterile conditions, they can survive for weeks as ‘organotypic slice cultures’. In both cases the visualization of the anatomy of the living slice helps locate electrodes, the mechanical stability greatly simplifies recordings from inside neurones, and the lack of a blood-brain barrier facilitates drug applications and changes in ion concentrations. Brain slices have proved immensely popular and successful, but it is important to remember that they are only one tool in the armoury needed to study brain function, and that ultimately results from them must be put in the context of the whole organism.
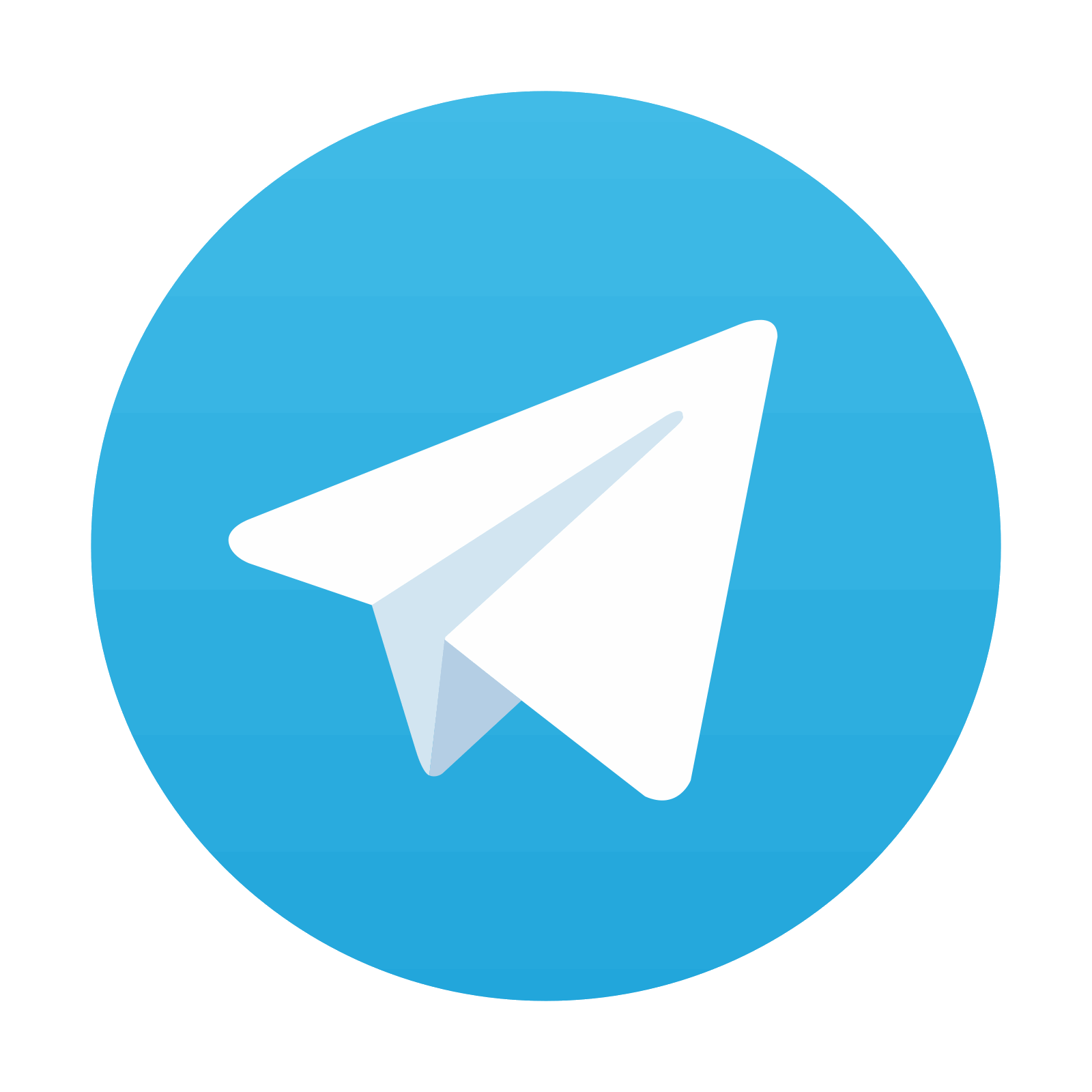
Stay updated, free articles. Join our Telegram channel
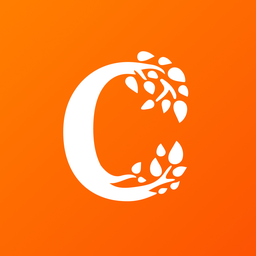
Full access? Get Clinical Tree
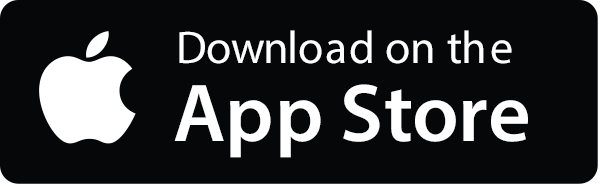
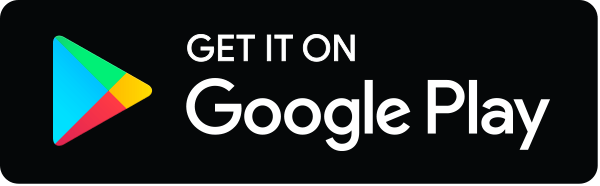