Systemic or intracerebroventricular
NMDA
Quisqualate
Kainic acid
Domoic acid
Pentylenetetrazol
Bicuculline
Allylglycine
Pilocarpine ± lithium
Soman
Flurothyl
Intracerebral
Kainic acid in amygdala
Dibutyl cAMP into amygdala
Folic acid into cortex
Penicillin into cortex
Bicuculline into cortex
Picrotoxin into cortex
Cobalt lesion + homocysteine
A disadvantage of chemoconvulsant models is that these agents themselves are toxic, and thus it is difficult to distinguish between their effects and those of the seizures. Furthermore, some of the agents such as bicuculline, picrotoxin, penicillin, kainic acid, and NMDA act on neurotransmitter receptors, which makes them unsuitable for studies aimed at understanding the alterations in the plasticity of neurotransmitter receptors during SE and for testing the novel therapeutic strategies which modulate inhibitory or excitatory neurotransmission or both.
Electrogenic models of SE were established to overcome the limitations associated with chemoconvulsants. Lothman and colleagues developed an electrical stimulation model of status epilepticus in which continuous electrical stimulation of the hippocampus triggers self-sustaining seizures that are restricted to the limbic system [47]. Electrical kindling of the amygdala or the stimulation of perforant path also produces SE [48, 49]. This stimulation model triggers neurodegeneration and the development of epilepsy, similar to that observed in pilocarpine or kainate models of SE.
Altered Neurotransmission in Status Epilepticus
A balance between excitatory and inhibitory neurotransmission maintains the neuronal firing rate, and a disruption of this homeostasis is associated with seizures. The γ-aminobutyric acid type A (GABA-A) receptor mediates the majority of inhibitory neurotransmission in the brain whereas ionotropic glutamate receptor subtypes, including NMDA, α-amino-3-hydroxy-5-methyl-4-isoxazolepropionic acid (AMPA), and kainate, contribute the majority of excitatory neurotransmission. The hippocampus plays an important role in the generation and sustaining of seizures, and it is a main structure affected by SE. Therefore, studies in animal models of GCSE have focused on understanding alterations in the neurotransmission of hippocampal dentate granule cells (DGCs) and CA1 pyramidal neurons during SE.
Impaired Inhibitory Neurotransmission
DGCs are highly inhibited, and their intrinsic excitability is much lower than that of hippocampal CA1 pyramidal neurons. DGCs are proposed to restrict spread of excitatory activity to neurons of the hippocampus proper, but impaired inhibition of DGCs could allow seizure spread into the hippocampus. The GABAergic inhibition of DGCs is compromised in animals in SE [50–52]; the frequency of synaptic currents is reduced, and their amplitude is also smaller. In addition, GABAergic inhibition of CA1 pyramidal neurons of animals in SE is also attenuated [53–55]. The entorhinal cortex provides substantial input into the hippocampus. Thus, these alterations likely allow activity from the entorhinal cortex to spread into the hippocampus and follow a reentrant loop that sustains seizure activity.
Biochemical studies have found reduced surface expression of γ2 subunit-containing GABA receptors, which are clustered at the synapses in the hippocampi of animals in SE [51, 55]. Development of resistance to benzodiazepines, which are the commonly used first-line therapy for SE, is a significant clinical problem that is also replicated in animal models of SE [41, 51, 56, 57]. Benzodiazepines exert their action via γ2 subunit-containing GABA receptors. The reduced expression of functional γ2 subunit-containing GABA receptors thus helps to explain a rapid reduction in the efficacy of diazepam to terminate SE [50, 53, 54, 58].
GABA receptors are constantly trafficked between the surface membrane and the intracellular pool. In a cell culture model of SE, repetitive action potential firing can be induced by incubation of cultures in a medium lacking Mg2+ ions or containing high extracellular potassium and NMDA. In these systems, the reduced surface expression of γ2 subunit-containing GABA receptors is associated with accelerated internalization of these receptors [51, 56, 59]. Similar to whole animal experiments, blocking the activity of protein phosphatases is sufficient to prevent the functional down-regulation of synaptic GABA receptors in cell culture models of SE [56]. Thus, preventing mechanisms that destabilize receptors at the surface membrane may provide targets to restore GABAergic inhibition.
Augmented Excitatory Glutamatergic Neurotransmission
Concomitant with reduced inhibitory neurotransmission, excitatory neurotransmission of hippocampal principal neurons mediated by NMDA and AMPA receptors is strengthened during GCSE [60]. There is internalization of GluA2 subunit-containing AMPA receptors during SE, which likely contributes to the calcium influx and activation of cellular signaling associated with cell death and epileptogenesis. The excitatory neurotransmission mediated by NMDA receptors is also augmented during GCSE [60]. Synaptic NMDA receptor-mediated currents recorded from DGCs of animals in GCSE were larger and had altered kinetics of decay. The cell surface expression of NR1 and NR2B subunits of NMDA receptors is also increased in the hippocampi of animals in GCSE.
Taken together, the dynamic movement of GABA receptors from the surface membrane toward intracellular compartments coincides with the insertion of NMDA and AMPA receptors into the surface membrane. Under normal conditions, mechanisms that maintain homeostatic synaptic plasticity ensure that when the network activity is enhanced, excitatory neurotransmission is downscaled and inhibitory neurotransmission is increased, such that the target firing rate is restored. Studies in animal models of SE, however, indicate that a failure in maintaining homeostatic synaptic plasticity contributes to prolonged seizures. If excitatory neurotransmission through AMPA or NMDA receptors is blocked, the seizures terminate [60–62]. NMDA antagonists also enhance the efficacy of diazepam, and a combination of NMDA antagonists and diazepam can be effective in terminating even prolonged SE [63, 64].
Histopathology of Experimental Status Epilepticus
Histopathologic investigations from electrogenic and chemoconvulsant models demonstrate patterns of cell loss identical to those in human GCSE. SE lasting for 30–60 min is sufficient to induce neuronal damage, and the severity of damage correlates with the duration of seizures [65]. Early immunohistochemical techniques, including Nissl and silver staining, have helped identification of gross morphologic changes and patterns of cell loss that occur following SE [37, 66, 67]. Subsequent studies that used fluoro-jade and TUNEL staining as well as expression of apoptosis marker proteins have provided additional insights into aspects of cell death triggered by SE [68].
Pattern and Evolution of Cell Death Following Status Epilepticus
The hippocampus is the region most susceptible to cell death following SE [41, 69, 70]. Extensive degeneration of CA3 and CA1 pyramidal neurons has been observed in different animal models of GCSE, whereas CA2 pyramidal neurons and DGCs are mostly preserved [65, 71–73]. In addition, middle layers of entorhinal cortex also suffer cell loss [74]. In these animal models, fluoro-jade, a marker of cell death, could be detected in hippocampal principal neurons as early as 3–4 days following SE. Additional cell loss may occur due to recurrent spontaneous seizures which appear following a seizure-free latent period of variable duration after SE.
GABAergic interneurons are also susceptible to neurodegeneration. Loss of inhibitory interneurons present in the hilus, particularly those expressing somatostatin, is observed in animal models of SE [75–78]. In contrast, parvalbumin- or cholecystokinin-positive interneurons are spared. This yields a complex modulation of inhibition, resulting in the net loss of the inhibitory inputs to the DGCs.
Mechanisms Underlying Neurodegeneration
It is reasonable to consider that systemic factors, such as elevated body temperature, and oxygen and glucose supply to the brain that are insufficient to cope with the increased demand of SE, may contribute to neurodegeneration. Cell death was still observed, however, in animals in which these parameters were maintained in the normal range during SE [83]. Instead, there is compelling evidence that glutamate-induced excitotoxicity plays a central role in cell death [84]. Canadian researchers reported that an acute neurologic syndrome, including seizures and SE, was due to ingestion of mussels from Prince Edward Island contaminated by domoic acid [85, 86]. Domoic acid is structurally similar to glutamate and probably activates glutamate-mediated excitation. Furthermore, blockade of NMDA receptors during experimental SE prevents cell death [87, 88]. Glutamate receptor-mediated calcium overload is likely to cause stress to mitochondria and endoplasmic reticulum, which also causes cell death [89, 90]. The breakdown of the blood–brain barrier during SE causes activation of glia and inflammation [91], which also appears to contribute to cell death.
Summary
The histopathologic damage induced by GCSE is straightforward based on human clinical, radiologic, and histologic studies and animal experimental data. Severe damage is limited to the specific fields of the hippocampus, and mild damage occurs in the cortex. Additional damage in the cerebellum, thalamus, and basal ganglia results from systemic physiologic alterations. Glutamate-mediated excitotoxic mechanisms play an important role in necrotic and apoptotic neuronal damage. Because neuronal injury is ongoing as long as seizure activity continues, patients who do not awaken immediately after treatment of SE must undergo EEG to exclude NCSE or they risk potential neuronal injury.
References
1.
Dodrill CB, Wilensky AJ. Intellectual impairment as an outcome of status epilepticus. Neurology. 1990;40(Suppl 2):23–7.PubMed
2.
Aicardi J, Chevrie JJ. Convulsive status epilepticus in infants and children. A study of 239 cases. Epilepsia. 1970;11(2):187–97.PubMed
3.
Lothman EW, Bertram EH. Epileptogenic effects of status epilepticus. Epilepsia. 1993;34(Suppl 1):S59–70.PubMed
5.
Hesdorffer DC, Logroscino G, Cascino G, Annegers JF, Hauser WA. Incidence of status epilepticus in Rochester, Minnesota, 1965–1984. Neurology. 1998;50(3):735–41.PubMed
6.
Eriksson KJ, Koivikko MJ. Status epilepticus in children: aetiology, treatment, and outcome. Dev Med Child Neurol. 1997;39(10):652–8.PubMed
7.
Gaspard N, Foreman BP, Alvarez V, Cabrera Kang C, Probasco JC, Jongeling AC, et al. Critical Care EEG Monitoring Research Consortium (CCEMRC). New-onset refractory status epilepticus: Etiology, clinical features, and outcome. Neurology. 2015;85(18):1604–13.PubMedPubMedCentral
8.
Hesdorffer DC, Logroscino G, Cascino G, Annegers JF, Hauser WA. Risk of unprovoked seizure after acute symptomatic seizure: effect of status epilepticus. Ann Neurol. 1998;44(6):908–12.PubMed
9.
Kramer RE, Luders H, Lesser RP, Weinstein MR, Dinner DS, Morris HH, Wyllie E. Transient focal abnormalities of neuroimaging studies during focal status epilepticus. Epilepsia. 1987;28(5):528–32.PubMed
10.
Nohria V, Lee N, Tien RD, Heinz ER, Smith JS, DeLong G, et al. Magnetic resonance imaging evidence of hippocampal sclerosis in progression: a case report. Epilepsia. 1994;35(6):1332–6.PubMed
11.
Tien RD, Felsberg GJ. The hippocampus in status epilepticus: demonstration of signal intensity and morphologic changes with sequential fast spin-echo MR imaging. Radiology. 1995;194(1):249–56.PubMed
12.
Wieshmann UC, Woermann FG, Lemieux L, Free SL, Bartlett PA, Smith SJ, et al. Development of hippocampal atrophy: a serial magnetic resonance imaging study in a patient who developed epilepsy after generalized status epilepticus. Epilepsia. 1997;38(11):1238–41.PubMed
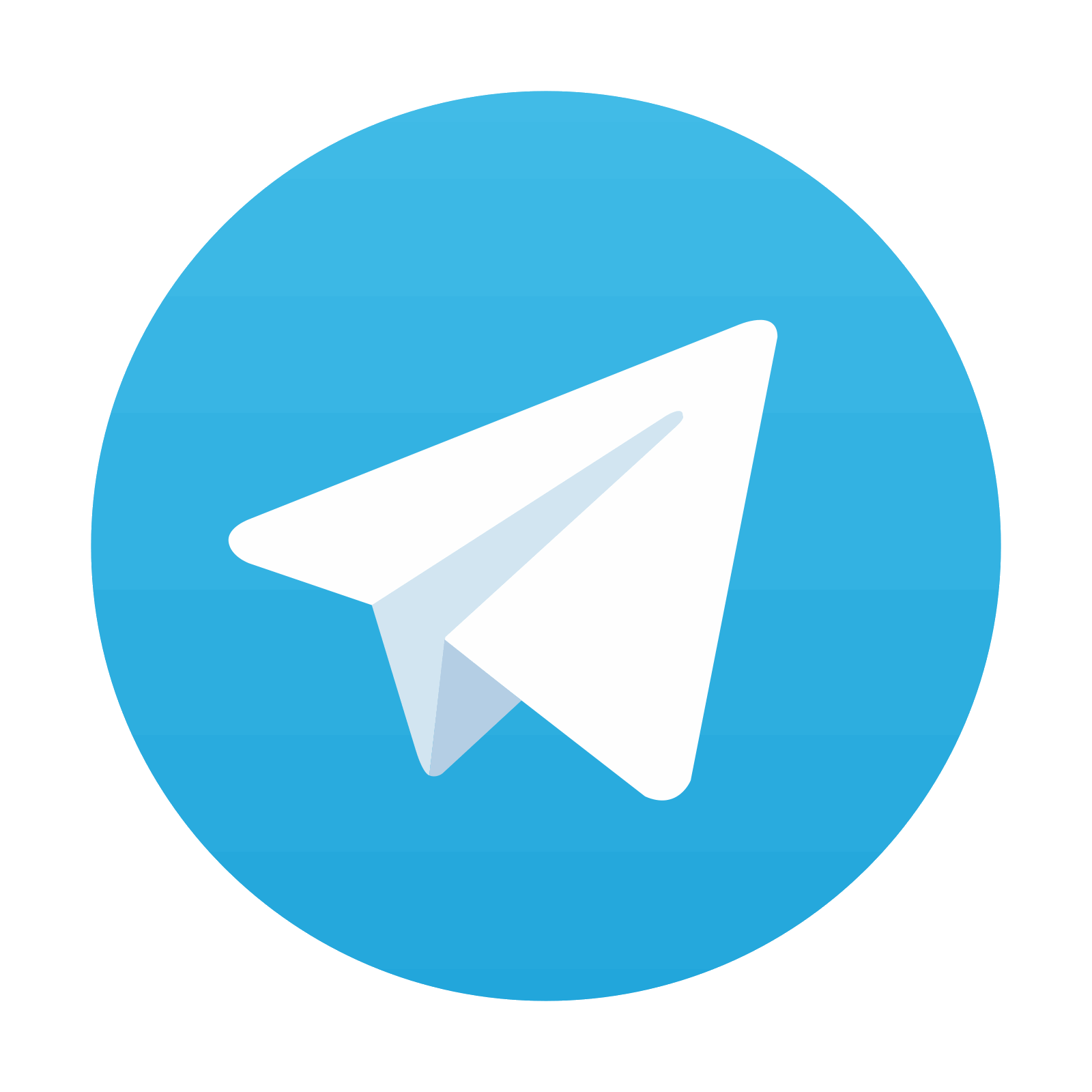
Stay updated, free articles. Join our Telegram channel
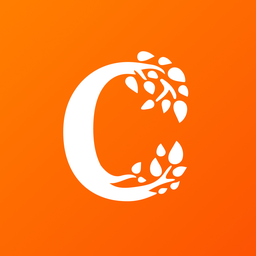
Full access? Get Clinical Tree
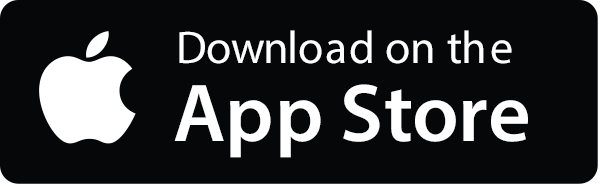
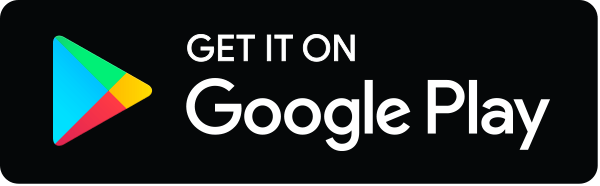