Fig. 24.1
Typical FES stimulation waveforms. (a) Rectangular pulse with exponential charge balance pulse. (b) Biphasic rectangular pulse. (c) Biphasic, rectangular pulse with reduced amplitude, but increased width of charge balance pulse
From a technical viewpoint, the exponential charge balance pulse (Fig. 24.1a) can be implemented in the simplest way by adding a series capacitor to the stimulation circuit. The generation of rectangular pulses is technically more complex but allows for a higher pulse repetition rate (= stimulation frequency in Hz) (Fig. 24.1b). In case of pulse widths below 300 μs, a short pause of approx. 100 μs is inserted between the stimulation and charge balance pulse. Without this pause the activation effect of the stimulation pulse is partly reduced due to the steep rise of the charge balance pulse [110]. If the amplitude of the charge balance pulse is substantially lower compared to the stimulation pulse (Fig. 24.1c), the pause can be omitted without any detrimental effect.
Basically, by FES an action potential can be triggered at any location of the nerve fiber – from the neuron via the axon to the motor end plate – which is then transmitted like in the physiological condition to the effector organ. It does not make a difference, if the stimulation impulses triggering the action potential are applied via surface electrodes, percutaneous wire electrodes, or fully implanted electrodes, which are places on a muscle or directly at the nerve’s axon.
Usually, for stimulation of innervated muscles, biphasic, charge-balanced rectangular impulses with a stimulation pulse width between 50 and 500 μs are used.
If innervated muscles are activated by electrically induced nerve action potentials, a relatively small amount of charge is needed (curve 4 in Fig. 24.2). A much higher charge is needed in case of direct stimulation of denervated muscle fibers, because the motor threshold of muscle membranes is 500–1000 times higher than the activation threshold of nerve membranes [5]. Even in the case of needle electrodes inserted directly into an innervated muscle, it is always the nerve and not the muscle fibers that are electrically activated. This is the reason why the term “muscle stimulation,” which is clinically often used in the context of innervated muscles, is misleading.


Fig. 24.2
Strength-duration curves of rectangular pulses for completely innervated (4.), decreasingly innervated (3., 2.), and completely denervated muscles (1.) [28]. The strength-duration curve shows the minimal current amplitude at a given pulse width, which is necessary to elicit a muscle twitch
With a larger extent of and longer time after denervation, more charge is needed to directly activate denervated muscles (curve 3 and 2 in Fig. 24.2). In case of a completely denervated muscle, impulses with high amplitude and pulse width need to be injected to generate contractions (curve 1 in Fig. 24.2) with the danger of exceeding the maximum permissible current density for surface electrodes of 2 mA/cm2 and the associated risk for local burns [50]. In any case, stimulated denervated muscles do not produce the same amount of force than innervated ones. Therefore, a careful screening for the presence of denervated muscles is mandatory to estimate the potential of a user for successful application of a neuroprosthesis. In Europe, a few stimulators are commercially available capable of generating short (<1 ms) impulses for stimulation of innervated muscles and long impulses (>100 ms) for direct muscle stimulation. With these devices, a precise testing of the innervation status of muscles and a training of denervated muscles to prevent muscle degeneration can be performed. Examples of such devices are the portable, single-channel Paresestim FES system from Medel GmbH, Hamburg, Germany, and the two-channel system stimulette den2x from Dr. Schuhfried Medizintechnik GmbH, Vienna, Austria.
The strength-duration curve for neural and muscle membrane flattens out with long stimulus durations, reaching an asymptote called rheobase. When the stimulus strength is below the rheobase, stimulation is ineffective even when stimulus duration is very long. The minimum pulse width needed to elicit a muscle contraction with a current of two times of the rheobase is defined as chronaxie. Both the rheobase and chronaxie are useful to document the degree of denervation and the course of reinnervation of a muscle.
Although electrically induced action potentials basically comply with physiological activation mechanisms, there are relevant differences between the physiological and artificial generation of muscle contractions [102]: In the physiological condition, if low muscle forces are generated, mainly thin fibers are activated. With increasing force, more thick fibers are recruited (Fig. 24.3). In case of external electrical stimulation, thick nerve fibers are first activated due to their higher electrical field and voltage gradient, and with increasing force, also smaller fibers get involved. However, thick nerve fibers end at fast-fatiguing muscle fibers, while thin fibers innervate more fatigue-resistant muscle fibers. This phenomenon is called “inverse recruitment” [82] and represents one of the main causes why stimulated muscles fatigue relatively fast compared to the physiological condition. In addition, all nerve fibers get activated nearly at the same time, which results in an almost synchronous activation of all muscles fibers of the corresponding motor units. Furthermore, each electrical impulse activates the same motor units. The inverse recruitment together with the synchronous activation of the same motor units causes a rapid fatigue of stimulated muscles. Basically, muscle fatigue occurs more quickly with higher rates of pulse repetition. Therefore, in practical FES applications, where tetanic contractions are needed, relatively low stimulation frequencies in the range of 16–20 Hz are used that allow for generation of a sufficient amount of force without having too much tremor. Nevertheless, continuous tetanic stimulation contractions at high force levels, e.g., needed for standing and walking, can only be generated over a short time period of 15–30 min depending on the amount of training. Additionally, the movements and forces generated by FES are less graduated when compared to the physiological condition. This is especially the case when low forces for fine control of hand and finger movements are needed.


Fig. 24.3
Recruitment of nerve fibers under physiological conditions and by electrical stimulation. Dark areas represent activated parts of nerves; light gray areas indicate inactive parts
In patients with chronic SCI, paralyzed muscles consist to a large extent of fast-fatiguing muscle fibers with the associated severely decreased fatigue resistance and capability for force generation. The disuse atrophy of paralyzed muscles can be reversed through FES training even many years after SCI. Additionally, FES training converts the fiber composition of muscles in the direction of slow fatiguing muscles [94].
24.3 SCI-Associated Conditions Limiting Neuroprosthetic Applications
In general, FES may not be applied in individuals with metal implants in the stimulated areas of the body or if active implants such as cardiac pacemakers or medication pumps are present. Besides these non-SCI-related exclusion criteria, a lot of secondary side effects associated with SCI may prevent the successful use of a neuroprosthesis. A thorough initial screening is mandatory to set up a realistic roadmap for each prospective neuroprosthesis user.
In individuals with paraplegia due to a lesion of the conus or cauda equina, damage to the lower motor neurons and/or their axons occurs. The presence of denervated muscles may prevent a successful application of neuroprosthesis. As stated before, stimulated denervated, flaccid muscles do not produce enough force to effectively contribute to a functional restoration sufficient for everyday use [50].
Individuals with a cervical SCI have a very heterogeneous neurological and functional status depending on the level, completeness, and type of lesion [11, 105]. Additionally, in about 30–50 % of individuals with tetraplegia, denervated muscles are present to a more or less extent due to the damage of motor neurons directly at the lesion site [26, 74].
Within the first 2 weeks after SCI, spasticity develops in many patients. Spasticity or clonus may be triggered by electrical stimulation in particular at the beginning of the FES training and each FES session. However, after some FES sessions, the muscle tone may be successfully reduced by the training [63].
If motor impairments persist, they lead to other negative effects. Immobility may lead to a reduction of the passive range of motion of affected joints, which may result in severe contractures with totally immobile joints due to calcified capsules. In such cases, even a strongly stimulated muscle is not able to generate joint movements. Intensive physical therapy may help to prevent some of these negative side effects on the musculoskeletal body structures.
In the chronic stage, profound disuse atrophy of the permanently paralyzed muscles is present. This may be reversed in innervated muscles by an FES-based training; however, if the SCI persists over a long time, it may take some months to achieve a fatigue resistance and force sufficient for neuroprosthetic applications [33].
Autonomic dysreflexia (AD) is a potentially dangerous clinical syndrome that develops in individuals with SCI, resulting in acute, uncontrolled hypertension. Briefly, AD develops within the first 6 months after injury in individuals with a neurologic level at or above the sixth thoracic level (T6). The occurrence of AD increases as the patient evolves from spinal shock. AD prevalence rates vary, but the generally accepted rate is above 50 % of all individuals with injuries at T6 and above. Patients with a sensorimotor complete injury have a much higher incidence of AD (91 % with complete injury vs. 27 % with incomplete injury) [22]. AD is caused by the damage of sympathetic spinal fibers and the resulting imbalanced innervation of the autonomous nervous system. Episodes of AD can be triggered by any strong stimulus below the level of injury [62]. AD may also be triggered by electrical stimulation of the lower extremity [4], but has also been seen by the author in very high lesioned patients during the application of a grasp neuroprosthesis.
Pain is a major problem after SCI and most of the patients report to have pain. In the acute phase after an SCI, it is mainly nociceptive pain due to trauma or spasm [30]. Usually within the first year after the injury, neuropathic pain develops in about 40–50 % of the patients and tends to become chronic [99]. Paresthesia in the stimulated dermatomes may restrict the application of a neuroprosthesis particularly systems based on surface electrodes.
24.4 Upper Extremity Neuroprostheses
Over the past 30 years, FES systems for restoration of upper extremity function with different levels of complexity and degree of invasiveness have been developed. While a handful of systems for restoration of a lost or restricted grasping function have reached a level of maturity sufficient for routine application in end users with tetraplegia [85], none is available for restoration of a lost reaching function suitable for everyday use.
24.4.1 Grasp Neuroprostheses
When using FES in a compensatory setup, the easiest way to improve a weak or lost grasp function is to apply multiple surface electrodes. Generally, their major advantages are their simple and safe application and that they can be offered to patients for temporary application at a very early stage of rehabilitation. During this phase the electrode setup has often to be adapted to the changing neurological condition due to spontaneous recovery. Additionally, costs of noninvasive systems are much lower than those of invasive neuroprostheses, and they can be applied by physical medicine and rehabilitation specialists without the need for surgical expertise [21]. Examples of noninvasive grasp neuroprostheses are (1) the commercially available NESS H200 System (formerly known as Handmaster, Bioness Inc., Valencia, CA, USA), which is mainly intended for training purposes, but can also be used as an assistive device for support of activities of daily living [1], and (2) the formerly commercially available Bionic Glove for improving the strength of a tenodesis grip in SCI individuals with preserved strong voluntary wrist extension [84]. Other more sophisticated prototypes in terms of control capabilities were successfully evaluated in the clinical setting, but are not available for home-based application [69, 106].
The main aim of the application of a grasp neuroprosthesis is to generate two grasp patterns, namely, the lateral grasp, also called key or pinch grasp, and the palmar grasp, also called cylinder or power grasp. The lateral grasp provides the ability to pick up flat objects between the flexed fingers and the flexing thumb, while the palmar grasp, in which the thumb is positioned in opposition to the index finger, permits larger objects to be handled. To generate these grasp patterns, electrodes on the thenar eminence and inside the hand are typically needed, which, however, tend to fall off or shift easily due to the extended skin and soft tissue movements in these areas [29]. However, with seven self-adhesive gel electrodes placed on dedicated positions on the forearm (Fig. 24.4a), the pinch and power grasp patterns can be restored without the need for electrodes on the hand [92]. The pinch grasp can be achieved by stimulation of the finger (ext. digitorum communis muscle, electrode pair (EP) 1 in Fig. 24.4a) and thumb (ext. pollicis longus, EP 2 in Fig. 24.4a) extensor muscles for hand opening, the finger flexors (flex. digitorum superficialis, flex. digitorum profundus) for hand closing and the thumb flexor (flex. pollicis longus) for grasping. In many SCI end users, it is possible to stimulate the flexor muscles of the fingers and the thumb with a common electrode pair (EP 3 in Fig. 24.4a). By implementation of a dedicated stimulation profile into a universally programmable, multichannel FES stimulator available from different vendors (e.g., Motionstim 8, Medel GmbH, Hamburg, Germany), it is possible to use co-contractions of the thumb flexor and extensor muscles to achieve a state, in which the fingers are sufficiently flexed and the thumb is still in an extended position. For the power grasp, a branch of the median nerve innervating the opponens pollicis muscle can be selectively stimulated with an electrode pair (EP 4 in Fig. 24.4a) placed on the medial side of the forearm.


Fig. 24.4
(a) Electrode positions together with the assigned channel numbers of the stimulator. Due to space limitations, channel 2 (ext. pollicis muscle) and channel 4 (opponens pollicis muscle) share a common electrode. (b) Electrodes fixed with a velcro strap in a personalized forearm sleeve for easy and quick electrode mounting. (c) Mounted forearm sleeve. To stabilize the wrist, a bent metal splint is integrated into the volar part of the sleeve
Apparent disadvantages of grasp neuroprostheses based on surface electrodes include difficulties with daily reproduction of the desired grasping movements and limited selectivity in stimulating deeper or small muscles. Additionally, patients consider the placement of the electrodes to be complicated [54]. Some of these limitations may be overcome by an adjunct fixation mechanism in the form of an orthosis or a sleeve. After the appropriate electrode positions have to be defined, a self-adhesive velcro strip is stuck on the top of the electrodes, which are then covered by a neoprene sleeve. This sleeve is manufactured according to the individual anatomy (proximal and distal diameter, length, position of the thumb) of the forearm (Fig. 24.4b). The velcro strips hold the electrodes in a fixed position in the sleeve, which dramatically reduces the time for donning. The sleeve contains a hole for the thumb and a mark sign at the proximal end, which facilitates the correct positioning of the sleeve on a daily basis (Fig. 24.4c).
As a precondition for a functional grasp, the wrist needs to be stabilized in neutral position during flexion of the fingers. In individuals with preserved elbow flexion, the wrist extensor muscle is often weak or denervated. In these cases, it is not possible to achieve a stable dorsal extension of the wrist by stimulation, and a fixation splint made of aluminum is integrated into the sleeve to maintain the wrist in neutral position during grasping. With this setup, a variety of activities of daily living (ADLs) can be performed by the users (Fig. 24.5).


Fig. 24.5
(a) Individual with cervical SCI and absent finger and thumb function writes with an ordinary ball pen. (b) Individual moves glass cylinder (120 g). (c) Eating a cookie. (d) Grasping a coin. (e) Holding a matchbox to light a match. (f) Manipulating a can
Another problem of noninvasive grasp neuroprostheses is the huge variation of the grasp patterns in dependence of the wrist rotation angle. In addition, defining the optimal size of stimulation electrodes during initial setup is often time-consuming. A promising concept for faster determination of the desired electrode positions and the dynamic compensation of position-dependent relocation of electrodes is the use of electrode arrays, in which small electrodes can be selectively activated and dynamically merged to virtual electrodes [41, 68, 83]. However, the clinical value of this newly introduced concept needs to be evaluated in future studies involving a larger number of end users.
Despite the technological progress made over the last two decades, some of the disadvantages of noninvasive neuroprostheses persist, among them are limited excitability of deeper muscle groups, painful sensations in case of paresthesia in the stimulated parts of the body, and handling problems. As a consequence, implantable neuroprostheses have been developed with different degrees of complexity: the BION [46, 67], a small single-channel microstimulator that can be injected through a cannula; a stimulus router system [31], an implantable electrode that picks up the current from surface electrodes; a multichannel implantable stimulator [100]; as well as a modular, networked, and wirelessly controlled system for stimulation and sensing [113]. In contrast to cardiac pacemakers, implants for restoration of complex motor functions need to deliver large amounts of energy to reanimate paralyzed limbs. This energy is typically provided through an inductive link either during operation (Freehand) or for charging batteries inside the implant (BION 3). Therefore, external coils need to be placed on the skin over the implant, and users of implanted motor neuroprostheses usually need to carry external components with them (Fig. 24.6c).
One of the implantable grasp neuroprostheses – the Freehand system – achieved commercialization in 1997 and was successfully used throughout the world by over 300 individuals with an SCI at C5/C6. It is therefore the most widespread implantable neuroprosthesis for restoration of the grasp function [47]. The first version of the Freehand system consisted of an implantable electrical stimulator, whose metal housing serves as a common anode (Fig. 24.6a, b), with eight stimulation channels and an external control unit, which contains the central processing unit and the batteries. In the early years, it used epimysial electrodes, which needed to be sutured to the muscle surface, but later it was possible to use intramuscular electrodes, which were directly inserted into a muscle and self-secured themselves with hooks. In the most recent revision, it is equipped with 12 stimulation channels and provides four channels for registration of myoelectric signals for control purposes [37].


Fig. 24.6
(a) X-ray of internal components of the Freehand neuroprosthesis in the right arm of an end user. (b) Implantable electrical stimulator with connectors, leads, and epimysial electrodes. (c) User eating with the Freehand system. The external shoulder joystick (left shoulder) and induction coil (right chest) are visible. The external control unit containing the microprocessor and batteries is not shown
The results of a multicenter trial including 51 Freehand users impressively demonstrated its high level of functional efficacy, end user satisfaction [80], and economic benefits [21]. Despite the proven clinical success, its commercialization stopped in 2001. This fact clearly demonstrates the difficulties industry encounters in sustained transfer of technology due to the small number of prospective end users and the few institutions providing the infrastructure for successful implantation of invasive neuroprostheses and for postoperative rehabilitation.
Besides their high costs, implantable systems inherently harbor the risk of infections and general surgery-related risks. Complex revision surgeries are necessary in case an implanted component fails. Although it has been shown that these events occur rather rarely [53], this risk has to be clearly communicated to patients who decide to receive an implant. Due to the fact that implantable neuroprosthesis and systems based on surface electrodes have distinct advantages and disadvantages, they represent alternative rather than competitive devices to surface electrode based systems.
Despite all the technical progress achieved, it has to be clearly stated that the degree of functional restoration by the currently available neuroprostheses based either on surface or implantable electrodes is rather limited. Even with the most sophisticated systems, it is only possible to restore one or two grasp patterns, and this does not include independent activation of single fingers or joints [52, 113]. Nevertheless, despite all their restrictions, grasp neuroprostheses represent a proven method for improving quality of life of end users with preserved shoulder function and elbow flexion, but missing hand and finger function.
24.4.2 Hybrid Neuroprosthesis for Grasping and Reaching
Most of the current neuroprostheses for the upper extremity are foreseen for individuals with preserved shoulder function and elbow flexion. In case of completely lost upper extremity function, meaningful restoration of a reaching and grasping function for activities of daily living by means of neuroprosthesis has not yet been demonstrated. The feasibility of using percutaneous electrodes for restoration of elbow extension and shoulder flexion/abduction was investigated only in an experimental setting [20, 45]. Recently, two advanced Freehand stimulators providing in total 24 simulation channels connected to either epimysial, intramuscular, or nerve cuff electrodes were successfully implanted in two individuals with an SCI above C4 [71]. Although shoulder, elbow, forearm, wrist, and hand movements were achieved in both subjects by this highly invasive and complex surgical approach, only one individual was able to perform two activities of daily living (scratching nose, shaking hands).
In general, a major problem in restoration of a completely lost upper extremity function is the rapid muscle fatigue of continuously stimulated shoulder and elbow muscles. To assist weak voluntary or stimulated shoulder forces, a mobile, passive, spring-based antigravity arm support system mounted on the wheelchair may be used [71, 81]. To avoid fatigue of stimulated muscles, so-called hybrid neuroprostheses consisting of a combination of FES and orthoses are proposed. In general, an orthosis is a mechanical device that fits to a limb and corrects a pathological joint function. An actively driven orthosis supports the joint movements with active electric or pneumatic actuators. The disadvantages of these exoskeletons are their mechanical complexity, limited possibility for home-based use in an ordinary everyday scenario, and their need for a sufficient power supply [96, 111]. Therefore, these systems are mainly intended to be applied in users for whom sufficient movements cannot be generated by FES.
If sufficient joint movements can be generated by FES, the application of an orthosis with an electromechanically un-/lockable joint is a more efficient solution than a robotic exoskeleton. In its released state, this joint allows for free movements and keeps a fixed joint position in the locked state. This locked state helps to avoid fatigue of the stimulated muscles needed to maintain a stable joint position. Both types of FES-hybrid orthoses may expand the group of prospective users of an upper extremity neuroprosthesis in the future.
At this point, it must be emphasized that the neurological status and functional capabilities of individuals with SCI, even with the same level of injury, vary to a large degree. As a consequence, an upper extremity neuroprosthesis necessarily has to consist of several modules that can be personalized according to the capabilities, needs, and priorities of an end user. Though this fact is well known among the neuroscience [9] and assistive technology community [44], very few technical solutions incorporate it [89].
For achieving a meaningful capability for object manipulation in individuals with an SCI at C4 or higher with only a few residual arm movements preserved, a mobile robot arm mounted on the wheelchair may be a cheaper, more robust, and better performing alternative than a full upper-arm neuroprosthesis based on FES. Some of these robot arms have been successfully tested in everyday scenarios in persons with tetraplegia, and some are commercially available, e.g., the iArm from Exact Dynamics, Didam, the Netherlands, and the Jaco arm from Kinova Robotics, Quebec, Canada. Whether these robotic arms are accepted by their users and an improvement of quality of life can be achieved in the long run, needs to be shown in future studies involving a larger number of end users.
24.4.3 User Interfaces for Control of Upper Extremity Neuroprostheses
The reliability and performance of the user interface of a neuroprosthesis have the same impact on the users’ acceptance as the meaningfulness of the FES-generated movements. Therefore, a robust user interface is a highly important component of a neuroprosthetic system. While users may be satisfied with a switch-based control scheme for step initiation of a lower extremity neuroprosthesis, the need for a sophisticated user interface for autonomous control of grasping is much higher in upper extremity neuroprostheses. The individual selection of the control method is mainly depending on the nature and degree of preserved functions under voluntary control of a user. The following functions may be used as command sources:
- 1.
Movements of mouth and tongue, including speech
- 2.
Voluntary movements or electrical activity of muscles not directly related to the function to be restored, e.g., movements or myoelectric activities of the contralateral shoulder or head movements
- 3.
Voluntary movements or electrical activity of muscles directly involved in the function to be restored, e.g., wrist extension or weak muscles of the forearm
- 4.
Specific cortical electrical activities
Speech control has not gained wide acceptance for neuroprosthesis control due to slow speed, long delays, and maloperation in noisy environments [77]. The use of tongue movements [43] or tooth clicks [32] may restrict the users’ ability to communicate. All user interfaces relying on mouth control are not appropriate if users wish to eat or drink with the help of the neuroprosthesis.
24.5 User Interfaces Using Functions Not Related to the Restored Movements
An established control method is the use of preserved movements not directly involved in the grasping function. Examples are the use of head orientation [115] or shoulder movements [100]. Command interfaces based on head movements or eye gaze may interfere with the user’s natural eye movements. These may differ substantially from those of healthy subjects, since users with SCI need to compensate the loss of tactile and proprioceptive feedback by visual feedback. Nevertheless, head movements or eye gaze may be applied successfully when combined with other input modalities such as preserved myoelectric activities of the shoulder [19].
Shoulder movements recorded by a two-axis shoulder position sensor were chosen as the standard input modality of the user interface of the implantable Freehand neuroprosthesis [100]. The degree of hand opening/closing and the force of the closed hand are normally controlled by the user through forward/backward movements of the contralateral shoulder in a proportional manner. Locking of the grasping position is initiated by a quick shoulder upward movement. An additional switch button is integrated into the shoulder joystick, which permits the user to switch between grasp patterns through a short press and the in-/activation of the entire system through a long press. In case shoulder movements are restricted due to muscular weakness or joint contractures, also electromyographic (EMG) signals from voluntarily activated muscles can also be used for control [38]. The performance of EMG- and movement-based control is at least for short-term use in the same range.
24.6 User Interfaces Using Activities of Muscles Directly Involved in the Restored Movements
For bilateral grasp restoration, the use of shoulder movements for neuroprosthesis control is limited, because shoulder movements are needed to place the hand correctly in space and, in so doing, interfere with the control function. An alternative involves using muscles that do not interfere with any upper extremity task. In some users, muscular functions below the level of injury, e.g., from the lower extremities, that are not functionally relevant may be used for control [73]. A promising way to set up a universal control interface involves selective activation of ear muscles [71, 109].
All of the control modalities mentioned above share the fact that the control is not “natural” for a user, i.e., it requires a substantial amount of training and special attention during use, particularly in the first weeks of use [38]. To overcome this limitation, the use of movements or muscle activities directly involved in the reaching or grasping function has been proposed. One of the first implementations of this approach was a proportional control of hand grasp by the degree of wrist extension measured either noninvasively [86] or by an implanted Hall-effect-based wrist angle sensor [8]. However, some users who are able to fully extend their wrist despite the presence of strongly stimulated finger flexors may not actually need a neuroprosthesis, if they use a natural tenodesis grip (passive flexion of finger and thumb flexors by active extension of the wrist) for everyday tasks.
A highly promising approach for a more natural and intuitive control involves monitoring the activity of weak muscles of the hand and arm. An example of this type of control is the use of the EMG activity of a weak brachioradialis or ext. carpi radialis longus muscle for proportional control of the grasp strength [51, 93, 106].
In individuals with high, complete SCI and the associated severe disabilities, too few residual functions may be preserved for setup of a reliable user interface. This has been a major limitation for the development of a reaching neuroprosthesis for individuals with a loss not only of hand and finger but also of elbow and shoulder function. Additionally, even end users who are basically able to use a certain interface may not be able to remain in control over an extended period of time due to physical and mental fatigue. Therefore, it is crucial for users to have a choice of options and for rehabilitation professionals to make them available, since each individual will find that some of the available options are more productive and work better than others. In this sense, brain-computer interfaces (BCIs) may serve as an alternative human-machine interface or may provide an additional control channel as an adjunct to traditional user interfaces.
24.7 Brain-Computer Interfaces for Control of Upper Extremity Neuroprostheses
BCIs are technical systems that provide a direct connection between the human brain and a computer. These kinds of systems are able to detect thought-modulated changes in brain activity and transform the changes into control signals. Most of the BCI systems rely on bioelectrical brain signals that are recorded noninvasively by electrodes on the scalp (electroencephalogram, EEG). A BCI system consists of five sequential components: (1) signal acquisition, (2) feature extraction, (3) feature translation, and (4) classification output, which interfaces to assistive devices and generates (5) a feedback to the user. These components are controlled by an operating protocol that defines the onset and timing of operation, the details of signal processing, the nature of the device commands, and the oversight of performance [98]. At present, EEG-based BCI systems can function in most environments with relatively inexpensive equipment and therefore offer the possibility of practical BCIs in end users’ home environment.
One type of EEG-based BCI exploits the modulation of sensorimotor rhythms (SMRs). These rhythms are oscillations in the EEG occurring in the alpha (8–12 Hz) and beta (13–30 Hz) bands and can be recorded over sensorimotor areas on the scalp. Their amplitude typically decreases during actual movement and similarly during mental rehearsal of movements (motor imagery, MI) [78]. It is known that people can learn to modulate the SMR amplitude by practicing MI of simple movements, e.g., hand/foot movements. This process occurs in a closed loop, meaning that the system recognizes, with the help of machine learning methods, the SMR amplitude changes induced evoked by MI, and these classification results are instantaneously fed back to the users. With the help of the feedback, users can adapt their mental modulation strategy to improve classification results. These neurofeedback-based procedure and mutual human-machine adaptation enable BCI users to control their SMR activity and use these modulations to control output device [15]. Although, most BCIs are used to generate binary control signals, MI-BCIs allow the detection of an intended movement based on brain signals, making them an exciting option for natural control of a grasping and reaching neuroprosthesis control, in particular in individuals with high SCI.
In the first pioneering work in BCI-controlled grasp neuroprosthesis, the BCI was used in a mainly digital manner (“brain switch”) as a substitute for the traditional neuroprosthesis control [76]. A novel development in BCI research is the introduction of the hybrid BCI concept [75]. A hybrid BCI (hBCI) consists of a combination of several BCIs or a BCI with other input devices. These input devices may be based on the registration of biosignals other than brain signals, such as EMG activities, or signals from traditional input devices like joysticks or mouses.
In a first single-case study, a combination of an MI-BCI and an analog shoulder position sensor was proposed [90]. With upward/downward movements of the shoulder, the user was able to control the degree of elbow extension/flexion or of hand opening/closing. The selection, if the analog signal from the shoulder position sensor is used for elbow or hand control, and the access to a pause state were determined by the brain switch provided by the MI-BCI.
With this setup, a highly paralyzed end user who had no preserved voluntary elbow, hand, or finger movements was able to perform several activities of daily living, among them eating a pretzel stick, signing a document, and eating an ice cream cone (Fig. 24.7), which he was not able to perform without the neuroprosthesis. He used short imaginations of movements of the right hand to switch from hand to elbow control or vice versa. A longer imagination led to a pause state with stimulation turned off or reactivated the system from the pause state.


Fig. 24.7
Sequence of pictures showing the eating of an ice cream cone with the support of a FES-hybrid orthosis in a user with completely lost elbow, wrist, and hand function (a). The user starts in the hand control mode and lifts his left shoulder to open the right hand for grasping the ice cone (b, c). After successfully grasping the ice cone, the user emits a BCI command to switch from hand control to elbow control and lifts his shoulder to flex his elbow (d). Now, the user licks the ice. Finally, the user lowers his left shoulder to extend the elbow on (e), he puts the cone in its original place and switches back to hand mode to release the ice cream cone on (f)
Despite research on the use of BCIs for neuroprosthesis control has seen tremendous progress in recent years, BCIs are not yet ready for independent home use. In general, setting up and handling current BCI systems is relatively complicated compared to traditional user interfaces and requires the (tele-)presence of technical experts. Thus, BCIs have to be improved to a stage at which end users together with their caregivers are able to apply the systems independently at home. A key component for achieving this goal is the availability of easier to handle, gel-less electrodes that provide a sufficient signal quality. Additionally, more studies with people in real need for a BCI such as individuals with high cervical SCI are needed to determine if research results obtained in able-bodied subjects also apply to neurologically impaired end users [91].
Initial human applications of invasive BCIs, in particular of intracortical electrode arrays for single-neuron recording, indicate that an intuitive brain control of robotic arms is possible without the need for extensive learning [17, 40]. However, this technology has not yet demonstrated its long-term stability over years. Additionally, due to the small number of study participants, the extent to which the results can be generalized remains unclear [103].
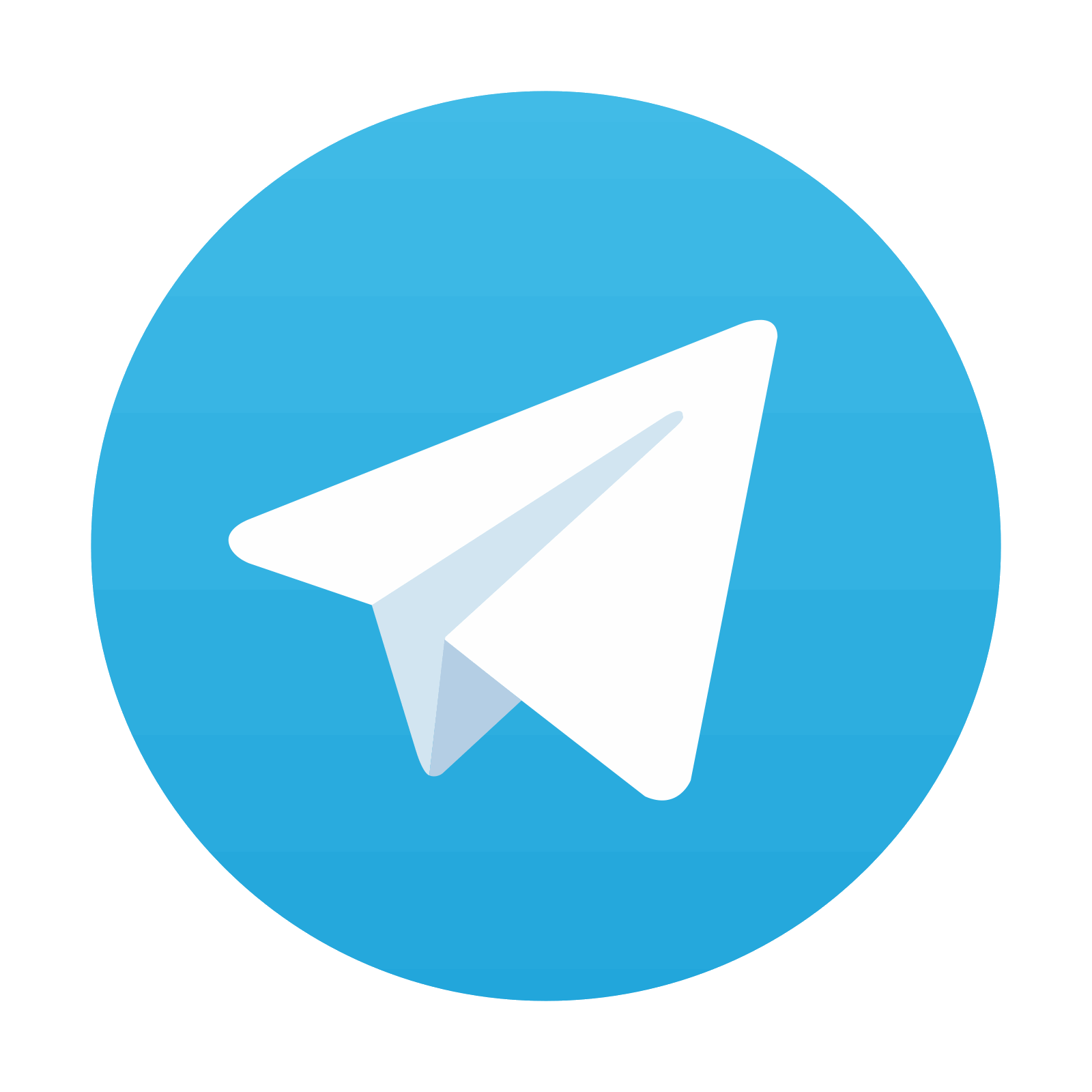
Stay updated, free articles. Join our Telegram channel
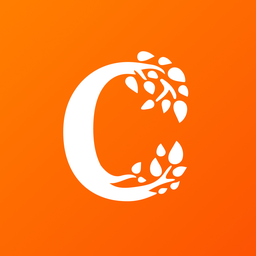
Full access? Get Clinical Tree
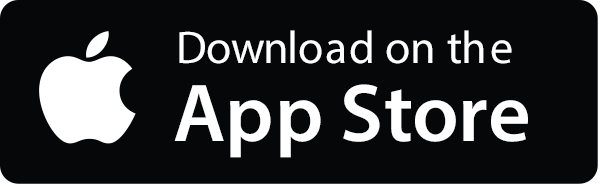
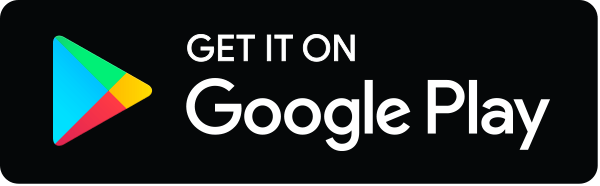