Traumatic brain injury.
A 42-year-old man presents to your intensive care unit (ICU) with acute brain trauma. He reportedly fell from a ladder at home while trying to fix the roof. There has been a loss of consciousness immediately after the fall, and he remains in obtunded mental status. In the emergency department (ED), his eyes open to painful stimulation, and he makes incomprehensible sounds and localizes to pain on the left side but is paretic on the right side (Glasgow Coma Scale [GCS] of 9: eye 2, verbal 2, motor 5). Pupils are reactive to light, and other brainstem reflexes are intact. The patient was intubated with an endotracheal tube. Initial vital signs: heart rate, 130 bpm; blood pressure, 160/90 mm Hg; oxygen saturation, 100% on assist control–volume control mechanical ventilation with FIO2 of 0.4; and tidal volume of 480 mL, at a rate of 12 times per minute. Body temperature is 37.5°C. A computed tomographic (CT) image of the brain without contrast is obtained (Figure 4-1).
This is a typical case of severe traumatic brain injury (TBI) with bilateral hemorrhagic contusions in the temporal lobes. Its proximity to the bony structures makes it a frequent location in the brain to be contused in trauma. Resuscitation of TBI patients varies widely, however, because of the heterogeneity of the disease itself. The aim of all good early resuscitation efforts is to begin as early as possible, with many efforts beginning in the prehospital setting, with an attention to airway, breathing, and circulation.
Three specific end points have been found to be independent predictors of poor outcome in the prehospital/ED setting: hypothermia, hypoxia, and hypotension.1 Hypothermia is likely a marker of poor resuscitation, and most would agree that core body temperature should be passively supported during the resuscitation phase rather than actively warmed with a device. Aggressive volume resuscitation for hypotension and adequate ventilation are the primary focus of initial resuscitation efforts. Prehospital resuscitation with hypertonic saline in TBI has failed to demonstrate a long-term benefit,2 and in a post-hoc analysis of the Saline versus Albumin Fluid Evaluation trial,3 fluid resuscitation with albumin was associated with higher mortality rates than was resuscitation with saline. Therefore, the administration of isotonic crystalloids is the preferred method by which to volume resuscitate. All TBI patients should be ventilated to a goal of normal partial pressure of carbon dioxide (Pco2) and be given supplemental oxygen to achieve Spo2 greater than 90%. During the early resuscitation phase, it is important to realize that simple measures such as elevation of the head of the bed (30°), midline positioning of the head (relieving any blockage of jugular venous drainage), and adequate pain control and sedation are very simple and effective methods to reduce intracranial pressure (ICP).
The patient is now admitted to an ICU, and the repeat CT of the brain 6 hours later confirms no expansion of the hemorrhagic contusion. The results of his neurologic examination remain poor, and he is only partially responsive to painful stimulation. Remembering that the literature reports the combination of severe TBI plus status epilepticus (SE) carries a high mortality rate, you wonder whether he could have had any nonconvulsive seizures.
Reported risk factors for seizures include a GCS score less than 10, cortical contusions, depressed skull fractures, wounds with dural penetration, prolonged length (> 24 hours) of coma, and posttraumatic amnesia. The majority of early posttraumatic seizures occur within the initial 48 hours of injury.4 However, some seizures may escape clinical detection and may be unnoticed in intubated, sedated patients in the absence of electroencephalographic monitoring. The presence of convulsive SE is associated with a high mortality rate. Effective prophylaxis of early posttraumatic seizures reduces brain metabolic demands, thereby reducing intracranial pressure and neurotransmitter release. This in turn minimizes secondary brain injury. Furthermore, anticonvulsant treatment can minimize cognitive and behavioral sequelae.
Phenytoin is an established standard antiepileptic drug (AED) in the setting of acute TBI. The American Academy of Neurology suggests using phenytoin for seizure prevention only in the first 7 days after TBI.5 It is important to remember that there is no added benefit of continuing it beyond 7 days if it was given as a prophylaxis in the setting of trauma. Although the availability of newer AEDs questions the use of phenytoin as the first-line AED in this setting, at this time there is no evidence to support an alternative to phenytoin for seizure prophylaxis in TBI. Levetiracetam has gained favor in acute brain injury setting because of its tolerability, ease of use without the need to follow a drug level, and minimal drug-to-drug interactions. It has been used in the neurocritical care setting for several years now, and numerous studies have reported on oral as well as IV use of this medication for the treatment or prevention of seizures in TBI. However, existing studies of levetiracetam regarding the safety and efficacy are not definitive and do not provide enough evidence that levetiracetam has better short- and long-term outcomes when compared with phenytoin.6
High doses of steroids are greatly beneficial in experimental models, reducing lipid peroxidation and improving tissue recovery. Clinical studies with glucocorticoids have not shown similar benefits. The results from the large Corticosteroid Randomization After Significant Head injury (CRASH) trial demonstrated no benefit and increased the mortality rate in TBI patients randomized to 3 g of methylprednisolone in the first 72 hours after injury. Currently, no data exist to support the use of glucocorticoid steroids acutely, and given the increased mortality rates seen in the CRASH trial, they are contraindicated acutely after brain injury.7,8
The Brain Trauma Foundation guidelines state that ICP should be monitored in those with a postresuscitation GCS of 3 to 8 and an abnormal CT scan and also for those with a similar severity and a normal CT scan if two of the following are present: age older than 40 years, posturing, or hypotension. The first choice for monitoring should always be an external ventricular drain because it can be recalibrated after placement and also offers therapy in the form of cerebrospinal fluid diversion. Parenchymal monitors provide continuous monitoring of ICP but can only be calibrated prior to placement and have an associated drift in their measurements.9
The ability to monitor brain tissue oxygen tension (Pbto2) has added a dimension of monitoring that provides insight to cerebral metabolism. The preponderance of case series data indicate that a Pbto2 level < 15 mm Hg is associated with poor outcome and that the patient may respond to either augmentation of mean arterial blood pressure (MAP), alteration in ventilation strategy, or transfusion of red blood cells. However, many questions regarding the utility of this monitoring technique have yet to be answered, such as timing, location, and pertinent treatment thresholds. Until such data become available, it is important to remember that the Pbto2 value is a composite of various components of the content of oxygen in the arteries as well as an unknown quantity of passive diffusion. This value is also a very focal/regional measure and should be put into context of the entire clinical picture prior to embarking on therapeutic interventions.10
There are sufficient data to support the use of periprocedural antibiotics, but the infusion must begin prior to the skin incision. The choice of antibiotic should be either cefazolin (1 g IV) or nafcillin (2 g IV). Vancomycin (1 g IV) can be utilized for those patients with a known penicillin allergy. The continued use of antibiotics for prophylaxis is controversial and has not been proven to reduce the rate of ventriculitis and may increase the incidence of drug-resistant bacterial infections.11
The 2007 Brain Trauma Foundation (BTF) guidelines have indicated that cerebral perfusion pressure (CPP) thresholds should be maintained within a range of > 50 mm Hg and < 70 mm Hg. This threshold is based on data that support the result that CPP values < 50 mm Hg are associated with more cerebral insults, whereas achieving CPP > 70 mm Hg for every TBI patient results in a fourfold increase in lung injury, likely due to aggressive volume resuscitation and excessive use of pressors.12 The use of Pbto2 monitoring may help guide targeted CPP management in order to avoid tissue hypoxia while minimizing the risk of complications related to pressure augmentation.
There have been two multicenter randomized controlled trials (RCTs) that have not demonstrated any benefit for the use of therapeutic hypothermia as a neuroprotectant.13-15 However, therapeutic hypothermia can still be considered as an option in the treatment of increased intracranial pressure.13 Owing to the lack of data and associated management complexities, therapeutic hypothermia has traditionally been reserved as a treatment option for patients who are either refractory or unable to receive osmotic therapy. The advent of modern temperature-modulating devices has allowed for a more safe and efficient delivery of therapeutic hypothermia, and as a result, this therapy may become more widely utilized in TBI patients. Because ICP is strongly dependent on core body temperature, any reduction in temperature to < 37°C will likely result in a decrease in ICP. However, this therapy has been traditionally targeted to lower core body temperature to 32°C to 34°C. The risk for infectious complications with therapeutic hypothermia is duration dependent, with the rate of infectious complications rising sharply at greater than 72 hours. Hypothermia will also result in a coagulopathy and increased risk of bleeding, although it is important to realize that there has not been any significant increase in intracranial bleeding due to hypothermia in any of the RCTs.
If hypothermia is utilized to control ICP, careful consideration should be also given to shivering, electrolyte imbalances, ventilator management, and rewarming. A commonly overlooked aspect of hypothermia’s effect on normal physiology occurs in relation to the management of ventilation and blood gas results. As a result of reduced metabolic activity, there is decreased production of carbon dioxide at lower body temperatures. If mechanical ventilation parameters are left unchanged during cooling, hyperventilation will occur, which may cause cerebral vasoconstriction and reduced cerebral blood flow. At lower temperatures, the solubility of gases in a liquid mixture increases. Most blood gas analyzers warm the sample to 37°C prior to determining the values for pH, Pco2, and Po2. Without correcting for the patient’s actual body temperature, this analysis will overestimate the Pco2 during hypothermia because portions of the dissolved gases come out of solution on warming and contribute to the partial pressure detected. Two methods of blood gas analysis exist with regard to changes in the patient’s body temperature: (1) alpha-stat management and (2) pH-stat management. Using alpha-stat ventilator management, the Pco2 detected by the blood-gas analyzer at 37°C is targeted to 40 mm Hg regardless of body temperature. This method of blood gas management may lead to hyperventilation as a result of the overestimation of Pco2. In contrast, pH-stat management targets a temperature-corrected Pco2 and pH. Using this method, as body temperature decreases, the total amount of CO2 in blood will increase as solubility increases. This poses the risk for inducing hypercapnia, which may result in cerebral vasodilation and increased intracranial pressure.16
In the middle of the night, the ICU resident calls to notify you that the patient’s left-side pupil has become enlarged (6 mm compared with 3 mm on the right) and is still reactive to light. The patient is right hemiplegic, and the left side is no longer localizing to pain. The overall mental status has been depressed further, and now painful stimulation does not lead to eye openings. Only minimal flexion to painful stimulation is seen in the left arm. Repeat CT reveals increased swelling and mass effect on the left temporal hemorrhagic lesion. ICP is 25 mm Hg, which is down from 40 mm Hg after 30 mL of 23.4% hypertonic saline injection given 4 hours ago. The patient is autohyperventilating, with a resulting end-tidal CO2 of 28 mm Hg. Licox monitoring shows Pbto2 of 16 mm Hg in the left hemisphere frontal subcortical region where the probe is positioned.
Surgical decompression limits the damage caused by secondary injury (delayed brain injury) by reducing increased ICP with subsequent improvement in brain oxygenation. As with any surgical procedure, there are inherent risks. The decision to perform decompressive techniques is primarily based on the evacuation of a mass lesion, with temporal and frontal lesions more likely to result in decompression. There are no standard clinical criteria, although many institutions reserve this procedure for younger patients in coma who have failed at least one ICP-lowering measure. The key is not to delay the procedure because the primary benefit is as a result of reducing sequelae from secondary injury. Once decompression is decided upon, resection of a larger bone fragment is performed to allow for greater dural expansion with a lower risk of herniation. All clinical studies of decompression have demonstrated immediate reductions in ICP; however, there is conflicting evidence regarding the effects of craniectomy on long-term outcomes. As a result, this technique is not as widely utilized as medical interventions for lowering ICP.17,18
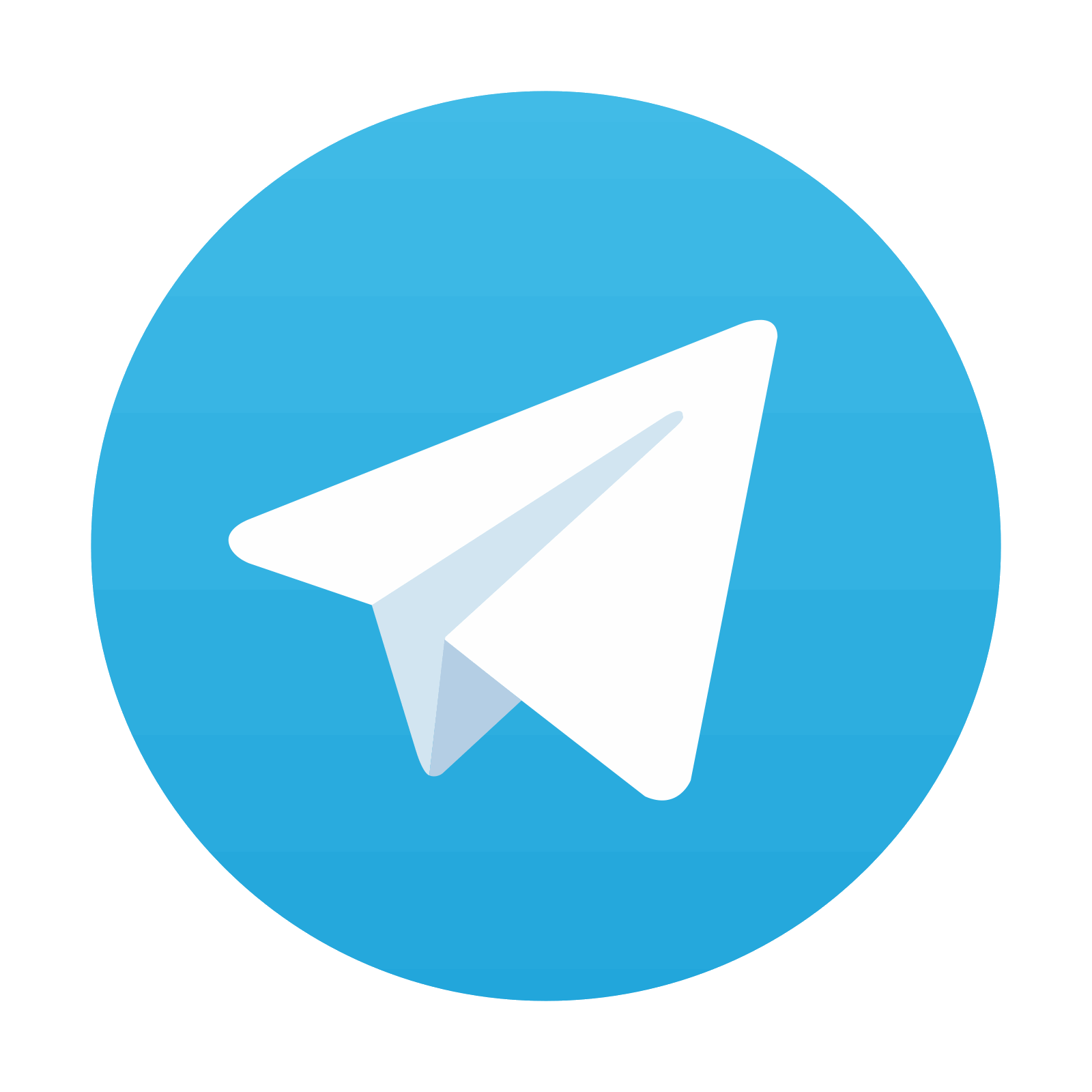
Stay updated, free articles. Join our Telegram channel
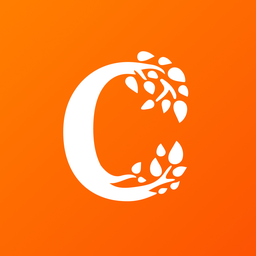
Full access? Get Clinical Tree
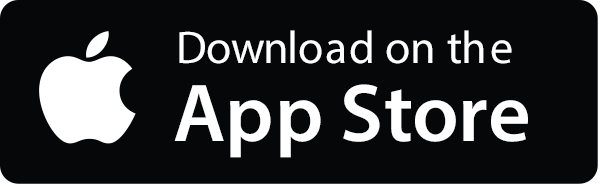
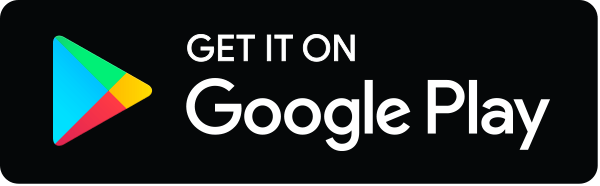