Fig. 9.1
The neurovascular unit. (a) Astrocytes are closely related to cerebral blood vessels and synapses. Astrocytes endfoot surround penetrating arterioles and fine processes are in close proximity to synapses. Activation of metabotropic glutamate receptors (mGluR) during synapse activity and propagation of Ca2+ waves from neighbouring astrocytes increase intracellular [Ca2+]. The vasodilation associated with neural activity could be promoted by astrocytic lipoxygenase products. (b) Microphotograph (400× magnification) showing glial fibrillary acidic protein (G-FAP) immunoreactive astrocytes surrounding the microvasculature in rat prefrontal cortex
Role of Vascular Endothelium
Once pial arteries penetrate into the brain they branch into smaller arteries and arterioles. These resemble peripheral vasculature, consisting of an endothelial cell layer, a smooth muscle cell layer (myocytes), and an outer adventitia layer, containing collagen, fibroblasts, and perivascular nerves [15]. The Virchow-Robin space, which contains cerebrospinal fluid, separates the vasculature from the glia limitans until arterioles and capillaries reach deeper into the brain and become smaller. Capillaries consist of endothelial cells, pericytes (with contractile properties), and the capillary basal lamina on which the astrocytic feet are attached [11].
The Endothelial Cells
Endothelial cells play a critical role in the regulation of both vascular tone and changes in the growth and morphology of the vessel wall by maintaining a vital balance between the various dilating and constrictor processes which influence many other cell activities. These actions are achieved by releasing diffusible factors such as nitric oxide (NO), free radicals, prostanoids, endothelium-derived hyperpolarizing factor, and endothelin [16]. Thus, endothelium cells are not only a simple monolayer barrier dividing different tissue, as they play an important role in several vascular events.
NO is produced following the conversion of l-arginine to l-citrulline by the catalytic action of the enzyme endothelial NO synthase (eNOS). This enzyme is constitutively expressed and its activity is modified by factors such as the shearing stress of flowing blood, bradykinin, and by exogenous application of acetylcholine. NO diffuses to stimulate soluble guanylate cyclase in vascular muscle, resulting in an increase in the intracellular concentration of cyclic guanosine monophosphate (cGMP), subsequent lowering of intracellular Ca2+ and muscle relaxation [16, 17]. NO also provides anticoagulant activity by inhibiting platelet activation as well as exerts anti-inflammatory effects, reducing vascular cell adhesion molecule 1 (VCAM-1) and intercellular adhesion molecule 1 (ICAM-1) expression and inhibiting release of chemokines, such as monocyte chemoattractant protein 1 (MCP-1) [17]. NO levels can be diminished either by its synthesis interference or by direct destruction when it reacts with superoxide anion. The reaction of NO with superoxide anion results in the formation of peroxynitrite, a potent oxidant that can produce cytotoxicity, including nitrosylation of proteins and damage to DNA [17].
Similar to NO, prostacyclin has vasodilator and anti-platelet aggregator functions and is viewed as an important agent of vascular protection. Prostacyclin is the prostanoid produced in the greatest amounts in the endothelium, which has enriched constitutive expression of cyclooxygenase (COX)-1. Prostacyclin receptor activation in muscle cells causes enhanced adenylate cyclase/cyclic adenosine monophosphate production resulting in vasodilatation. Additionally, under pathological microenvironmental conditions, in addition to other prostanoids, prostacyclin can be generated by COX-2 isoform [17].
The Blood−Brain Barrier
Brain endothelial cells from capillaries are unique because they are not fenestrated and are sealed by tight junctions, features that underlie the BBB. The combined surface area of these microvessels constitutes the largest interface for blood–brain exchange by far. The surface area, depending on the anatomical region, gives a brain exchange total surrounding area between 12 and 18 m2 for the average human adult [18].
Its particular characteristics provide a stable environment for neural function and synaptic activity by (1) controlling ion movements; (2) keeping separate pools for central and peripheral transmitters, minimizing cross-talk; (3) preventing the entrance of many circulating macromolecules; (4) protecting neurotoxic substance to reaching brain tissues; and (5) allowing passive transport for many essential nutrients and metabolites [18].
The peculiarity of BBB is given by the specific phenotype of the endothelial cells, with adherences junctions as cell–cell interaction stabilizers, and tight junctions (TJs) that reduce permeation. The latter limit the paracellular flow of water, ions, and larger molecules into the brain (gate function) from the blood plasma to the brain extracellular fluid, and organize the cell membrane in apical–basal domains (fence function). The extreme effectiveness of the TJs in the impediment if ion movement results in the high in vivo electrical resistance observed for the BBB [18, 19].
The current statement is that under normal circumstances the tightness and transport properties of the BBB are relatively constant, which is in agreement with the need for a conserved extracellular milieu allowing normal central nervous system (CNS) functioning. However, many of the cell types associated with brain microvessels, including microglia and astrocytes and nerve terminals adjacent to the endothelial extracellular matrix/basal lamina release vasoactive agents and cytokines that can modify TJs assembly and barrier permeability [18, 19].
Role of Astrocytes
Direct contact between central neural terminals and vascular smooth muscle is infrequent and a process of extracellular diffusion of vasoactive agents released from active synapses in the brain parenchyma to local arterioles cannot account the temporally coordinated vascular changes that underlie CBF increases. In this sense, functional hyperemia cannot be explained solely by an interaction between neurons and local vessels, other cells must also be involved [10].
Gray matter astrocytes (protoplasmic astrocytes) have a polarized anatomical structure and entail two types of processes: fine perisynaptic processes that cover most synapses, providing optimal support for synaptic transmission; and large diameter vascular processes (end feet) that are near in the vicinity of the vessel wall, covering >99 % of the vascular surface facing endothelial cells or pericytes [10]. Furthermore, astrocytes can produce a great variety of vasoactive substances such as NO, ATP and cyclooxygenase, and epoxygenase activity derived products [20].
The first proposed mediator was potassium ions (K+). As modest increases in extracellular K+ concentration can hyperpolarize smooth muscle cells, it was postulated that K+ released from active neurons depolarized astrocytes, leading to K+ efflux from astrocyte end feet [3].
Nevertheless, evidence accumulated over the years has led to the proposal of a different main mechanism, taking into account Ca2+ signaling in astrocytes. These ion oscillations are known to be restricted to microdomains in the processes of individual astrocytes and are observed when synaptic activity occurs [20]. Furthermore, stimulation of neuronal afferents, direct mechanical stimulation of individual astrocytes and mGlu-receptor agonist were found to trigger temporally correlated Ca2+ elevations in astrocyte end-feet and dilation of cerebral arterioles [21]. Neuronal activity-dependent dilation of cerebral arterioles was reduced either when Ca2+ oscillations evoked in astrocytes by synaptic glutamate were inhibited using mGlu-receptor antagonists, or when COX inhibitors that block prostaglandin synthesis were used. These results are further validated as in vivo records of blood flow in the somatosensory cortex by laser Doppler flowmetry. The hyperemic response evoked by forepaw stimulation was markedly reduced after the systemic application of mGluR antagonists [21]. Additionally, subsequent experiments indicate that vasodilation of the microvasculature could be mediated, at least in part, by prostaglandin E2 (PGE2), because astrocytes in culture were observed to release this powerful dilating agent in a pulsatile manner according to the pattern of mGlu-receptor stimulation mediated Ca2+ oscillations [22].
A model is then proposed where astrocytes can encode different levels of neuronal activity into defined Ca2+ oscillation frequencies that, at the level of perivascular end feet, mediate the release of COX-derived vasoactive agents. The prostaglandins, mediating physiological vasodilation in response to a rise in astrocyte Ca2+, are mainly produced by COX-1, which is expressed in astrocyte. Neuronal activity-dependent Ca2+ oscillations may ultimately represent the signaling system that allows blood flow to vary in a manner proportional to the intensity of neuronal activity [20] (Fig. 9.1).
However, in pathological conditions, expression of COX-2 is upregulated in astrocytes and this might also contribute to prostaglandin synthesis. Considering this new information, it is now given to understand the role of the different isoforms of the enzymes that synthesize the vasoactive messengers [3].
Vascular Pathologies and Cognitive Impairment
Endothelial Dysfunction
The endothelium plays a central role in the regulation of vascular tone through timely and balanced production and release of endothelial relaxing factors, as well as endothelium-derived contracting autacoids, and also by detection of diverse physical, chemical, or mechanical stimuli. Moreover, healthy endothelial cells continuously adapt to local requirements and are essential for the maintenance of the entire vascular homeostasis involving antioxidant, anti-inflammatory, profibrinolytic, and anticoagulant effects, in addition to trophy effects in surrounding cells [23].
Endothelial dysfunction is primarily characterized by the loss of NO bioavailability and contingent with elevated levels of reactive oxygen species (ROS). These events have far-reaching implications, not only because the imbalance promotes smooth muscle cell activation, which leads to proliferation and migration; but also upregulates the endothelial expression of adhesion molecules, which induces immune cell recruitment to the vascular wall. Inflammation, in turn, enhances oxidative stress by upregulating the expression of free radical-producing enzymes and by downregulating antioxidant defenses. Finally, endothelial dysfunction is also characterized by increased production of endothelium-derived contracting factors. All these events are involved in an impaired regulation of vascular tone [23–26].
Oxidative Stress
Oxidative stress in the vasculature appears to be a common feature in diverse models of cerebral vascular disease and injury. This pathological state occurs as the result of an imbalance that favors the generation of ROS over its metabolism by various antioxidant defense mechanisms. There are multiple sources of the main ROS, superoxide, in the vasculature; including mitochondria, nicotinamide adenine dinucleotide phosphate (NADPH) oxidase, COX, and xanthine oxidase. Cerebral endothelial cells are metabolically active and the number of mitochondria present in cerebral endothelium is high, thus generating high levels of superoxide during oxidative phosphorylation. The effects of ROS are prominent in the cerebral circulation because cerebral blood vessels have the capacity to generate high levels of superoxide and are particularly sensitive to the effects of ROS. Low concentrations of ROS function as mediators and modulators of cell signaling. By contrast, higher levels of ROS commonly contribute to vascular disease. The overall effects of ROS depend on local concentrations, subcellular localization, and the proximity of ROS to other target molecules [7, 25].
Vascular Effects
Superoxide can have direct effects such as reacting with enzymes containing iron–sulphur centers resulting in release of free iron and subsequent formation of the highly reactive hydroxyl radical. Additionally, the formation of multiple other biochemical species such as superoxide radical, hydrogen peroxide, and reactive nitrogen species is dependent on production of superoxide [7, 25].
Besides responses that are dependent on endothelial cells, other vasodilator mechanisms are inhibited by ROS. Similarly to endothelium-dependent vasodilation, neurovascular coupling is also impaired by superoxide. Reductions in resting blood flow and impairment of vasodilator responses as a result of oxidative stress may result in a mismatch between energy requirements, substrate delivery, and clearance of cellular by-products [7, 25]. Furthermore, oxidative stress attenuates the growth factor support provided by endothelial cells to oligodendrocyte precursors. Loss of trophy support may impede the proliferation, migration, and differentiation of oligodendrocyte progenitor cells and compromise the repair of the damaged white matter [26].
Many studies have shown that ROS affect vascular structure or growth. ROS might produce hypertrophy by inactivation of NO that normally inhibits vascular growth, or through direct activation of signaling cascades involved in growth of vascular muscle including growth factors, kinases, and transcription factors. Such structural changes can have functional consequences because remodeling and hypertrophy also contribute to the shift in autoregulation by reducing the vascular lumen and increasing cerebrovascular resistance. Alterations in autoregulation increase the susceptibility of the brain to cerebral ischemia when blood pressure drops because cerebral blood vessels fail to compensate for the reduction in perfusion pressure [10].
Blood Brain Barrier: Alterations
Superoxide or other ROS increase permeability of the BBB. Dysfunction of endothelial cell-to-cell junctions, which disrupts the endothelial barrier and increases vascular permeability, appears to be also involved in the pathogenesis of vascular failure [24, 27]. As senescence animal show increased levels of oxidative stress, it has been proposed that ROS that are locally produced in brain parenchyma can trigger alterations of the BBB, possibly by cell death, gliosis, and signaling changes. In aged Wistar rats, which show impairment in short term-memory, leakage through the BBB was found to be associated with microglial activation, which are a known source of oxidative damage. Leakage of BBB can induce microglial activation by letting abnormal molecules pass into the brain parenchyma and in turn free radicals released from the microglia may further alter the BBB, in a vicious cycle leading to perivascular edema and axonal demyelination. Demyelination slows the transmission of nerve impulses and might contribute to the neural dysfunction. More importantly, in a rodent model of senescence, morphological changes of BBB and leakage of endogenous albumin and immunoglobulin G (IgG) to the brain parenchyma were selectively demonstrated for brain regions involved cognition, such as the hippocampus [19, 26].
Vascular Cognitive Impairment
In the past years the idea that the cerebrovascular dysfunction often precedes the onset of cognitive impairment has been extensively debated, taking into account that alterations that disrupt the homeostasis of the cerebral microenvironment could promote the neuronal dysfunction underlying the impairment in cognition. Structural and functional integrity of cerebral blood vessels is vital for the preservation of cognitive function. To a great extent, cognitive health depends on cerebrovascular health [11, 26, 28].
Vascular cognitive impairment (VCI) refers to the broad spectrum of cognitive deficits associated with cerebrovascular diseases. In 2011, The American Heart Association/American Stroke Association presented a statement to define and understand the rationale behind the use of the term VCI [29]. There has been significant growing terminology to characterize the cognitive syndrome associated with risk factors for cerebrovascular disease and its manifestations, especially in the description of dementia. In the past years the term vascular dementia (VaD) has been used, regardless of the pathogenesis of the vascular lesion—ischemic or hemorrhagic or single or multiple infarct (s). Moreover, cerebrovascular disease can affect multiple cognitive functions and vascular mild cognitive impairment (VaMCI) has been proposed as the “vascular” equivalent of the known mild cognitive impairment. In this context, VCI encompasses all the cognitive disorders associated with cerebrovascular disease, from frank dementia to mild cognitive deficits. Thus, VCI is a syndrome with evidence of clinical stroke or subclinical vascular brain injury and cognitive impairment affecting at least one cognitive domain, VaD being the most severe form of VCI [29].
Vascular-related structural pathology may produce so-called vascular dementia, including multiple infarcts, hemorrhagic events, focal infarcts, subcortical white matter disease or hemispheric ischemia, and hemorrhagic stroke [30–32]. Beyond the impact of these fixed structural lesions, hemodynamic dysfunction of CBF, which includes hypoperfusion and altered cerebral autoregulation, may be independently associated with cognitive decline. Hemodynamic effects may occur at the level of large vessels at the neck or head, at a global level in the setting of cardiac failure, or intrinsically as a result of dysfunction of the endothelium in the microvasculature [33].
Currently, all diagnostic criteria to characterize cognitive syndromes associated with vascular disease should be based on two factors: demonstration of the presence of a cognitive disorder (dementia or VaMCI) by neuropsychological testing and history of clinical stroke or presence of vascular disease by neuroimaging that suggests a link between the cognitive disorder and vascular disease. Clinical studies have shown that subjects with VaMCI can present a broad cognitive impairment, which can also include memory deficits [29]. Patients with VaD show mild impairment of memory with impairments in executive function such as judgment. Those who have memory problems tend to have more difficulty with encoding new information, thereby limiting acquisition rather than the pattern of rapid forgetting seen in Alzheimer Disease (AD), which is related primarily to poor retention. In order to determine the relationship of cerebrovascular disease to the cognitive symptoms, it is critical to identify the presence of cortical or subcortical infarcts or other stroke lesions with neuroimaging, and these should be associated with clinical symptomatology. It may also be important to consider the source of the cardiac or vascular pathology that underlies the cerebrovascular disease associated with VCI to provide a more specific clinical pathologic relationship [29, 32, 34].
It is important to highlight that after AD, VaD is the second most common form of dementia, comprising 10–20 % of all dementias. Data suggest that the annual incidence of VaD may range from 20 to 40 per 100,000 in people between 60 and 69 years of age to 200–700 per 100,000 in people over 80 years of age, with prevalence rates doubling every 5 years. As with cerebral ischemic disease, the risk of VaD is somewhat higher for men than it is for women [32].
Additionally, there is a decreased survival rate for patients with VaD as compared with patients with AD, presumably related to underlying cardiovascular disease risk factors. Risk factors for VaD reflect the general risk factors associated with cerebral ischemia: age, hypertension, diabetes, obesity, cigarette smoking, hyperlipidemia, and cardiac disease (including coronary artery disease and cardiac arrhythmias) [32, 35]. Association of vascular risk factors with cognitive decline and dementia are probably mediated largely by cerebrovascular disease, which can have additive or synergistic effects with coexisting neurodegenerative lesions [36].
Brain Renin–Angiotensin System Modulates Vascular Events: Therapeutic Opportunities
The Renin–Angiotensin System
The classical effects of the renin−angiotensin system (RAS) were related to its endocrine role in the electrolytic homeostasis and control of the blood pressure. Angiotensin II (ANGII) acts by rapidly increasing vascular resistance and through long-term effects acting on vasculature, heart, kidney, sympathetic output, and the CNS, promoting vasopressin and aldosterone actions and regulating thirst and water intake [37–40].
Several receptors have been identified to be activated by ANGII, among them the AT1 receptor (AT1-R), which is the one mediating most of the ANGII physiological and pathological functions. This surface receptor belongs to the G-protein-coupled receptor family and has a seven transmembrane domain conformation [40–42]. AT1-R activates multiple intracellular signaling pathways. Mainly, it promotes inositol trisphosphate (IP3) formation and Ca+2 releases from intracellular compartments, adenylcyclase inhibition, modulation of voltage-dependent Ca+ channels, or activation of phospholipase C (PLC). Secondary pathways involving mitogen-activated protein kinase (MAPK), extracellular-signal-regulated kinase (ERK) or c-jun N-terminal kinase (JNK) activation have also been described, this way participating in trophy events. Independent G-protein activation pathways involve later desensitization and even internalization by endocytosis mediated by β-arrestins [40].
Over the years it has become apparent that a local autocrine or paracrine RAS may exist in a number of tissues, implying new roles for this system [43]. Moreover, the functions of the tissues and systemic activities show significant differences. Even though circulating ANGII levels may not be intensively high, AT1-R expression in different organs is abundant enough to promote intracellular signaling. Furthermore, locally produced ANGII concentration may be higher than plasma levels and elicit a response in tissues with relative low AT1-R expression [40]. In particular, brain microvessels have all of RAS components (precursors, enzymes, and receptors) indicating the presence of a local RAS [44]. This evidence suggests that cerebral circulation is particularly sensitive to ANGII. Cerebral blood vessels are exposed to ANGII from both circulating and local (formed locally within the vessel wall and brain) sources, which play an active role in cerebrovascular events. There is much evidence supporting the idea that most ANGII effects occur independently of the effects of ANGII on arterial pressure [45, 46].
Renin–Angiotensin System and Oxidative Stress
Activation of ANGII AT1-R leads to activation of protein kinase C (PKC), which in turn phosphorylates p47 phox, leading to the assembly of the enzyme and ROS production. ANGII also increases vascular expression of components of NADPH oxidase and levels of superoxide. Contributions of ANGII to oxidative stress are due in large part to activation of NADPH oxidase. ANGII-induced endothelial dysfunction is prevented by inhibition or genetic deletion of AT1-R, pharmacological scavengers of superoxide or genetic deletion of NADPH-oxidase isoform 2 (Nox 2) [7, 12]. Spontaneously hypertensive rats (SHR), an animal model for studying hypertension, display elevated levels of all of RAS components in brain microvessels and allow the evaluation of ANGII-action overexpression [44]. These animals present a role switch in cerebrovascular NOS isoenzymes, from physiologic and protective, to deleterious. While overproduction of NO by the inducible form (iNOS) promotes neurotoxicity, the diminished production of NO by the eNOS alters regional blood flow. The imbalance observed in NO roles is partly a consequence of AT1-R activation, given that blockade of these receptors restored the normal proportions of NOS isoenzymes. The final result obtained is that eNOS activation is restored, and vasodilatation and reversed pathologic arterial remodeling is improved. Furthermore, iNOS inhibition reduces ANGII-induced ROS production, NO scavenging, cellular damage, and inflammation [47].
A key feature of oxidative stress is that once it is initiated, ROS or peroxynitrite can feed forward, promoting additional oxidative stress. RAS may be one of the mechanisms that contribute to these effects. Oxidized angiotensinogen is more readily cleaved than the non-oxidized form. Thus, in an oxidative environment, the oxidized form of angiotensinogen can be more prevalent. In this form, ANGII-induced oxidative stress may feed forward, promoting further production of ANGII [7].
RAS and Neurovascular Coupling
Evidence relating to ANGII and neurovascular coupling first indicated that ANGII does not affect the neural processes that generate the vasodilator response, but inhibit their vascular effects, resulting in attenuation of the vascular response associated with neural activation. Systemic administration of ANGII elevates medial arterial pressure (MAP) and attenuates the increase in CBF produced by whisker stimulation in the somatosensory cortex. Nevertheless, this ANGII-induced attenuation of functional hyperemia is independent of blood presssure elevation, as still persists even when the elevations in MAP are offset by removal of small amounts of arterial blood or if the systemic effects of ANGII are prevented by direct application of the peptide to the somatosensory cortex. However, the effects of systemic ANGII on functional hyperemia were not associated with attenuation of the field potentials evoked by whisker stimulation in the same area [46].
Consequently, much evidence suggests a role for ROS in this pathological role of ANGII. The attenuation of functional hyperemia induced by acute or chronic (7 days) administration of ANGII can be reversed by ROS scavengers, genetic deletion, or pharmacological intervention of NADPH-oxidase subunits. All of these manipulations also reverse ANGII-induced ROS production in these vessels. Moreover, it was found that NADPH-oxidase subunits and AT1-R are present in the same endothelial cells and in close proximity [48]. In mice lacking the NADPH oxidase subunit Nox-2, nonselective NOS inhibition or selective inactivation of eNOS prevents the ANGII-induced extensive nitration of cerebral blood vessels. These findings provided new evidence suggesting that the effects of ANGII on CBF responses to acetylcholine and whisker stimulation also requires peroxynitrite, which is formed mainly from eNOS-derived NO and Nox-2-derived superoxide [11]. Endothelial function is greatly impaired in a genetic model of chronic ANGII-dependent hypertension via a mechanism that involves increased oxidative stress. The mechanism which accounts for this dysfunction involves superoxide, as treatment with polyethylene glycol superoxide dismutase (PEG-SOD) completely restored vascular responses [49].
Further studies have demonstrated that the cerebrovascular oxidative stress and the attenuated endothelium-dependent response induced by systemic administration of ANGII can be blocked by AT1-R inhibition, free radical scavengers, NADPH oxidase peptide inhibitors, and is not observed in Nox-2-null mice [49].
The vascular dysregulation induced by ANGII also requires constitutive levels of COX-1-derived prostaglandin E receptor type 1 (PGE2) acting on EP1 receptors in order to achieve NADPH oxidase-dependent ROS production. Based on the localization of COX-1 in microglia and of EP1 receptors in cerebral arterioles, PGE2 could originate from microglia and act on vascular EP1 receptors to enable ANGII-induced vascular oxidative stress [50].
All of the above-mentioned findings support the concept that cerebrovascular oxidative stress mediates the powerful effects of ANGII on the cerebral circulation, which may contribute to the susceptibility to ischemic injury and dementia associated with hypertension [50].
RAS and Inflammation
Two key mechanisms appear to underlie the classical ANGII-induced inflammatory response: (1) generation of ROS and (2) production of nuclear transcription factor kappa-B (NF-κB) [17].
As previously assessed, ANGII is a powerful modulator of ROS production in endothelial cells and vascular smooth muscle cells. The ANGII-induced oxidant stress by AT1-R activation is closely associated with elevation of agents such as VCAM-1, ICAM-1 and MCP-1 and thus initiation and progression of vascular inflammation [17]. In SHR, increased endothelial AT1-R correlates with increased ICAM-1 expression, higher numbers of endothelium-adhering macrophages in cerebral microvessels and carotid artery, and an increased number of perivascular infiltrating macrophages in microvessels [51]. Chronic infusion of ANGII at the slow-pressor dose has been shown to cause significant increase of leukocyte adhesion on brain venules at the same time it promotes oxidative stress development [52, 53]. Moreover, systemic injection of ANGII in rats produces arteriolar leukocyte adhesion and increases P-selectin, E-selectin, ICAM-1 and VCAM-1 expression in arterioles and venules [53]. However, it is not entirely clear if enhanced ROS generation is the primary event in ANGII-mediated vascular dysfunction or if the oxidant stress and inflammatory response act synergistically [17].
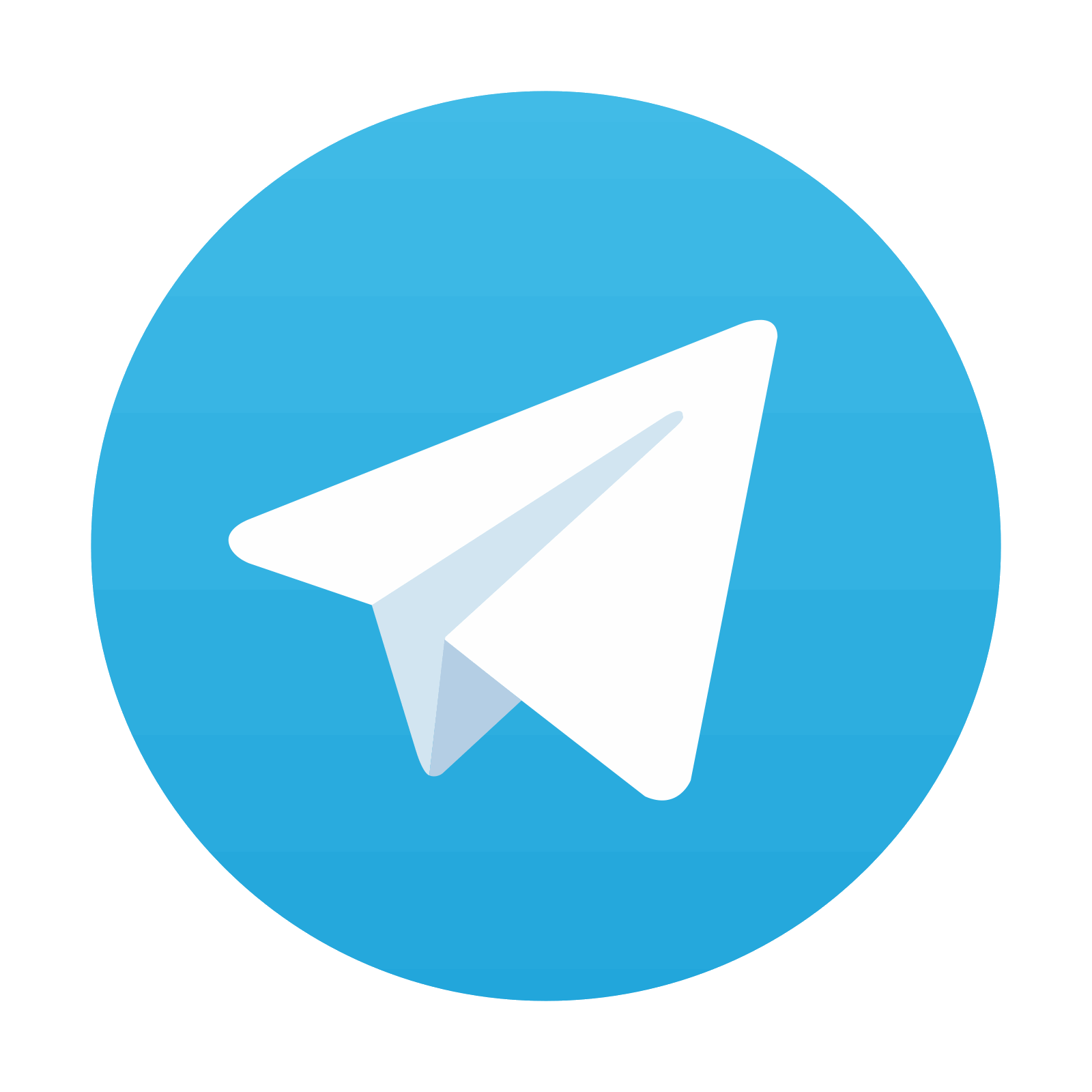
Stay updated, free articles. Join our Telegram channel
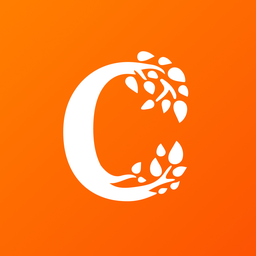
Full access? Get Clinical Tree
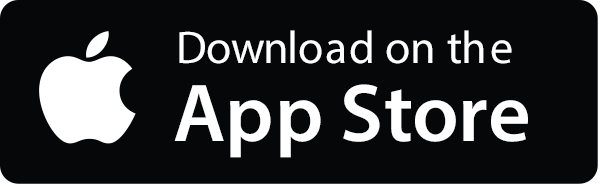
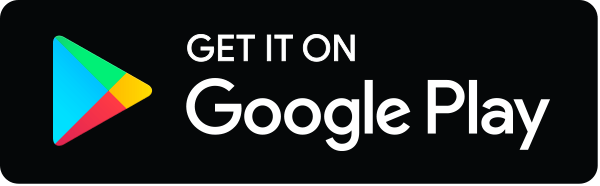