New Frontiers in EEG: High and Low Frequencies, High-Density EEG, Digital Analysis, and Magnetoencephalography
David E. Burdette
Andrew Zillgitt
Since the early days of electroencephalography (EEG), neurophysiologists have attempted to identify and characterize the dynamic nature of the normal and abnormal fluctuations in electric fields produced by the brain. Early attempts were hindered by cumbersome equipment and a paucity of channels for simultaneous recording. With improvements in instrumentation, increasing numbers of channels were available for simultaneous recording. Since the 1960s, the standard for recording scalp EEG has utilized the 10-20 system of electrode placement first proposed by the International Federation of Clinical Neurophysiology in 1958,1 and the bulk of scalp EEG literature has focused upon visual interpretation of electrocerebral potentials falling within the 0.5- to 30-Hz range and recorded using the 10-20 system.
Realizing that visual inspection of the EEG recordings using the 10-20 system has limitations with regard to spatial and frequency representations, neurophysiologists have expanded the limits of electrocerebral recording in a number of ways. This expansion has benefitted from advances in computer technology and increasing memory storage capabilities. This chapter will focus on these frontiers of EEG. Specifically, we will cover four areas: (1) frequency bands outside of those typically analyzed on scalp EEG, (2) digital EEG signal analysis, (3) high-density EEG recording, and (4) magnetoencephalography (MEG, an alternative method of visualizing the dynamic, ionic fluctuations within the brain).
ATYPICAL FREQUENCY BANDS
A number of recording constraints determine the frequency response that will be present in the EEG. These constraints include the sampling rate in digital EEG, the responsiveness of pen deflection in analog EEG, the low-frequency (high-pass) filter, and the high-frequency (low-pass) filter. By selecting appropriate recording parameters, the neurophysiologist can preferentially evaluate the high- or low-frequency spectrum of the recorded EEG.
As noted in previous chapters, routine scalp EEG records fluctuations in electric fields produced by the summation of large numbers of excitatory and inhibitory postsynaptic potentials (EPSPs and IPSPs, respectively). Also discussed in previous chapters is the onerous presence of noncerebral electric fields that fluctuate within the vicinity of that frequency range. EEG electrodes passively detect all electric fields impinging upon them, so these noncerebral sources can obscure the electrocerebral activity that we are trying to record. Further impeding our ability to interpret electrocerebral activity is the limited frequency range represented on routine scalp EEG. Low-frequency activity (<0.5 Hz) and high-frequency activity (>30 Hz) occur prominently within the brain and may yield valuable information about disease processes. We discuss below the rationale, potential benefits, and drawbacks of recording outside of the typical frequency bands.
REVIEW
20.1: All of the following represent new frontiers for EEG recording EXCEPT:
a. Analog EEG recording
b. Digital signal analysis of EEG activity
c. Higher-density EEG electrode placement
d. Magnetoencephalography
e. High-frequency and very low-frequency recordings
View Answer
20.1: a. Analog EEG was the mainstay of EEG recordings from the 1920s through the 1990s but is seldom seen now due to ubiquitous use of digital EEG recording systems.
Low-Frequency/Infraslow Activity
As detailed in Chapter 1, the electrocerebral activity recorded on scalp EEG results from electric fields produced by ionic flow into and out of neurons produced by depolarization (EPSPs) and hyperpolarization (IPSPs) of the dendrites oriented
orthogonally to the brain surface. Summation of large numbers of these neuronal ionic shifts produces the majority of the electrocerebral potentials routinely seen on scalp EEG. Most scalp-EEG recordings assess electrocerebral activity that is fluctuating at 0.5-30 Hz; however, there is a wealth of slow (<0.5 Hz) and infraslow (<0.01 Hz) activities that can be recorded using nonpolarizing (silver-silver chloride) electrodes and EEG machines equipped with special DC amplifiers.2
orthogonally to the brain surface. Summation of large numbers of these neuronal ionic shifts produces the majority of the electrocerebral potentials routinely seen on scalp EEG. Most scalp-EEG recordings assess electrocerebral activity that is fluctuating at 0.5-30 Hz; however, there is a wealth of slow (<0.5 Hz) and infraslow (<0.01 Hz) activities that can be recorded using nonpolarizing (silver-silver chloride) electrodes and EEG machines equipped with special DC amplifiers.2
In electronics, the abbreviation “DC” typically denotes “direct current.” Direct current here refers to current that does not oscillate (eg, the current that flows from a battery). In EEG, the abbreviation “DC” actually stands for two different things, the direct current involved in the slow shifts in recorded EEG potentials and the “direct coupled” amplifier that allows recording of those shifts.
The term “DC shift” refers to the slow “shifts” in potential that accompany infraslow EEG activity. This term is a bit of a misnomer because unlike DC current in the world of electronics, infraslow EEG activity does fluctuate; nevertheless, the terms infraslow EEG activity and DC shifts are largely interchangeable.
The second electroencephalographic way in which we use the term “DC” refers to “direct coupling,” which describes the amplifier configuration that is ideal for recording infraslow activity. Amplifiers on typical EEG machines have four stages. These stages control sensitivity, low-frequency filtering, high-frequency filtering, and notch (50 or 60 Hz) filtering.3 The low-frequency filter uses a capacitor that has a characteristic time constant to attenuate low-frequency activity. That capacitor allows large voltages relative to ground to dissipate (with a time constant) so that the small oscillations superimposed on the absolute potential from ground can be amplified without saturating the amplifier. Hence, the low-frequency filter prevents either overloading of the EEG amplifier or production of EEG fluctuations that exceed the range of the pen on analog EEGs (thereby causing “pen blocking”). When one is careful to avoid system overload, recording with a “direct coupled” amplifier is possible, with no capacitor (low-frequency filter) stage of amplification, that is, the sensitivity and high-frequency filter stages are “direct coupled.” Nonpurists can review slow EEG activity at the upper end of the infraslow range by setting the time constant of their reading software to the highest setting. For instance, a time constant of 2 seconds roughly corresponds to a low-frequency filter setting of 0.08 Hz.
The origin of infraslow activity or DC shifts seen on EEG recordings may be artifactual or cerebral. Artifactual causes of infraslow activity include environmental artifacts such as slow body movements with or without electrode swaying as well as bioelectric artifacts including slow eye movements, tongue movements, and sweatassociated artifact. When evaluating DC EEG, one must be aware of potential artifactual causes of DC shifts. Fortunately, assessing the presence of a believable cerebral field is the same for infraslow activity as for routine EEG. For instance, potentials that exhibit triple phase reversals on bipolar montages or any phase reversal (in the absence of a contaminated reference) on referential montages are likely to be of artifactual origin.
Brain Generators of Infraslow Activity/DC Shifts
The origins of infraslow EEG activity include neuronal sources, glial sources, and the blood-brain barrier.4 Neuronally generated infraslow activity may occur when a sustained volley of incoming EPSPs and IPSPs produces a longer lasting shift in ion concentrations inside and outside the dendrite. Sustained neuronal excitation typically produces increased intracellular sodium and extracellular potassium. This situation is likely to occur during an electrographic seizure. Infraslow activity of glial origin can also occur in any situation in which there is a buildup of extracellular potassium. Glial cells help maintain ionic homeostasis by actively transporting potassium into the glial cell, thereby producing a slow DC shift. Most situations in which there is an excess of extracellular potassium (eg, ischemia, seizure activity, or spreading cortical depression [SCD]) will result in glial cell-driven infraslow activity on DC EEG. Recordings from glial cells have demonstrated propagating, spontaneous slow oscillations in the infraslow range.5 Finally, situations that increase or reduce the potential difference between blood and cerebrospinal fluid (CSF) (hyperventilation, hypoventilation, and changes in cerebral blood flow) across the blood-brain barrier may produce infraslow potentials.4
Slow and infraslow activity may be focal or diffuse, and when analyzing this activity, the EEG interpreter must visually assess the activity to assure that it exhibits a believable cerebral field. Processes that affect both cerebral hemispheres in a diffuse fashion will produce diffuse changes on the EEG, and analysis of infraslow activity is no exception. The CO2 and pH changes that accompany respiratory effort produce infraslow activities that are diffuse and driven by the electrochemical gradient across the blood-brain barrier.4 Hypercapnia produces infraslow positivity, and hypocapnia generates prominent infraslow negativity.6 Changes in cerebral blood flow and cerebral blood volume produce diffuse, midline-maximum slow potentials via effects on the blood-brain barrier.7 Vertex-maximum, diffuse, infraslow EEG activity also occurs in normal sleep, particularly during non-REM sleep in association with arousals (positive polarity shifts) and shifts into deeper sleep stages (negative polarity shifts).8
Ictal Infraslow Activity
Infraslow electrocerebral activities occur in brain regions that are involved in seizure activity. Work in the 1960s indicated that absence seizures were associated with diffuse, infraslow activity (Fig. 20.1).9 More recent data have demonstrated that focal infraslow activity can be helpful in determining the laterality and focality of seizures recorded with intracranial depth electrodes (Fig. 20.2). Focal infraslow activity has been identified in the region of seizure onset up to 3 hours before ictal onset, and the power of infraslow activity is strongly biphasic with a peak at seizure onset and at seizure offset.10,11 Epidurally recorded seizures, however, failed to identify reliably timed or localized ictal infraslow activity.12 Scalp-EEG recordings have revealed a prominent, negative polarity shift with temporal lobe seizures that lasted for the
duration of the seizure.7 These DC shifts occurred in the temporal derivations and agreed with the side of ictal onset. These scalp and intracranially recorded EEG studies indicate that ictal DC shifts may yield complementary information regarding the site(s) of seizure onset, but larger series are needed to define better the role of DC EEG in seizure localization.
duration of the seizure.7 These DC shifts occurred in the temporal derivations and agreed with the side of ictal onset. These scalp and intracranially recorded EEG studies indicate that ictal DC shifts may yield complementary information regarding the site(s) of seizure onset, but larger series are needed to define better the role of DC EEG in seizure localization.
Evoked Infraslow Activity
DC shifts are an important component of cognitive and motor evoked potentials. One method to assess brain activity associated with cognitive planning involves presenting two stimuli, S1 and S2, such that S1 (the first stimulus) serves as a warning that the second paired stimulus, S2, is coming. When S2 occurs, the subject is to perform a cognitive or motor task. Approximately 200-500 ms after S1, an infraslow DC shift occurs called the contingent negative variation (CNV).13 The initial components of the CNV consist of frontally dominant negativity. Similarly, motor planning is associated with an infraslow DC shift. This can be seen during DC recording of self-paced repetitive motor activities such as finger tapping.14 Approximately 500-1000 ms prior to each movement is a negative polarity, vertex-maximum DC shift called the Bereitschaft potential. Both the CNV and the Bereitschaft potentials may be of low amplitude and are best viewed using averaging techniques.
Infraslow Activity and Migraine
Particularly illustrative of the benefits of DC recording is the story of SCD (of Leão). SCD is a slowly propagating (typically 2-6 mm/min) wave of suppression of neuronal activity.15 This was originally observed and described in the early 1940s by Leão, who discovered the phenomenon while studying an animal model of seizures in anesthetized rabbits by electrically stimulating the brain. Instead of the expected seizure, he instead found that stimulation would produce a slowly spreading wave of depressed cortical activity.16 Due to limitations in EEG technology, the original descriptions of SCD were based upon standard, low-frequencyfiltered EEG recordings. Subsequent DC recordings have demonstrated that this slowly spreading wave of depressed cerebral activity is accompanied by a prominent, negative-polarity DC shift. Further DC EEG as well as neuroimaging techniques have demonstrated that SCD is actually a spreading cortical depolarization, since a rim of increased neuronal activity is at the leading edge of the prominent infraslow DC shift.17 The DC shift is thus the result of disruption of ionic homeostasis with accompanying (temporary) loss of normal neuronal function (ie, the spreading “depression”). Hence, DC EEG revealed that the spreading wave of depressed neuronal function was, in fact, due to a prominent, negative polarity DC shift caused by loss of ionic homeostasis.18
SCD has been implicated as the neuropathologic cause of the migraine aura (Fig. 20.3) and transient global amnesia and may explain some of the clinical phenomena that accompany head trauma and cerebral ischemia.19,20 DC recordings will likely be at the forefront of neurophysiological characterization of these events and may provide an objective means of evaluating both the ictal (SCD) features of migraine and “interictal” migrainous phenomena. In addition to the prominent DC changes associated with SCD, high-frequency “gamma range” activity also occurs in the leading edge of the SCD in animal models and may prove to be important in developing new treatments for migraine.21
REVIEW
20.2: Which of the following is NOT true about infraslow EEG recording?
a. DC recordings are directly coupled to the amplifier without low-frequency filtering.
b. DC potential shifts can result from shifts in extracellular K+ concentration.
c. Infraslow activity can help localize ictal onset zones during seizure activity.
d. The Bereitschaft potential is a frontal positivity associated with motor planning.
e. Spreading cortical depression in migraine is associated with negative DC shifts.
View Answer
20.2: d. The Bereitschaft potential is a frontal negativity associated with motor planning.
High-Frequency Oscillations
When one considers that inhibitory and excitatory postsynaptic potentials last 20-100 ms and that scalp EEG is recording the summation of large numbers of these postsynaptic potentials, one would expect to see electrocerebral potentials fluctuating at 10-50 Hz (ie, one postsynaptic potential every 0.02-0.1 seconds) or faster when large volleys of these potentials occur asynchronously.22,23 Additionally, action potentials, though not evident on scalp EEG, can be recorded with electrodes placed within or near the brain (eg, from depth, subdural, or epidural electrodes); volleys of synchronous action potentials lasting 1-2 ms would produce even higher-frequency EEG fluctuations.22 Finally, gap junctions—the 2-nm pores that electrically couple the intracellular space between adjacent cells—have been shown to play a role in some high-frequency oscillations (HFOs) at 200 Hz.24 The evaluation of electrocerebral activity >30 Hz is limited on scalp EEG by the highfrequency filtering effects of the skull as well as inadequate pen response in analog systems.23 A simple example of higher frequencies that are evident on scalp-EEG recording is the “breach rhythm” that occurs in the presence of a skull defect. The breach rhythm consists of faster frequencies that would ordinarily be filtered by the underlying skull (Fig. 20.4). Faster frequencies of cerebral origin will not be evident on visual inspection of the scalp EEG except in the following circumstances: (1) the presence of a breach in the skull, (2) seizures originating in a large volume of neocortex, (3) intracranial recordings, and (4) evoked potentials in which nonsynchronized higher-amplitude, slower frequencies have been averaged out, thereby leaving only the evoked higher-frequency activities.
For the purposes of this chapter, the term HFO means frequencies >30 Hz. Various names for high-frequency bands have been suggested, but none has gained wide acceptance except for the term, “gamma range.” Gamma range activity was proposed for activity of 30-80 Hz although there has been some variation in this definition, even expanding the frequency band to 25-400 Hz.25,26 Because the nomenclature remains in evolution, EEG investigators have simply described the higher-frequency bands that they have identified or investigated in numerical terms.
The significance of electrocerebral activity within the typical alpha, beta, delta, and theta ranges has been well established through empirical observation since the days of Hans Berger, but the significance of HFOs is less well understood. As with electrocerebral activity in most frequency bands, HFOs may be normal or abnormal. Evaluation of normal HFOs and the effect of various disorders on them has been an area of interest to cognitive neuroscientists, as will be discussed below. The evaluation of abnormal HFOs has been of particular interest to epileptologists, which is not surprising since (as noted above) they are the result of rapid, synchronous neuronal activity.
Recording Parameters
As with the recording and interpretation of slow activity, one must use appropriate recording parameters for HFOs. These include adequate sampling rates and the judicious use of filters. The sampling rate must be at least twice the highest frequency that is being sampled in order to avoid aliasing,23 and if an accurate characterization of the morphology is desired, then even higher sampling rates should be used. Ideally, one should record EEG data in a DC fashion without filters and view without filters as well. However, the presence of artifact and the tendency for the amplitude of lower-frequency electrocerebral activity to overwhelm that of the higher frequencies (thereby masking them) often makes the use of filters necessary. As when viewing any EEG, one should initially view the data with minimal filtering and then review the recorded signals with necessary adjustment of filters. When focusing on higher-frequency activity, it may be necessary on the second review to reduce the time constant of the low-frequency filter significantly in order
to attenuate slow frequencies and to focus on HFOs, which may be buried in the slower activities.
to attenuate slow frequencies and to focus on HFOs, which may be buried in the slower activities.
As with the interpretation of any electrocerebral activity, the interpreter must always be cognizant of the possibility of artifact. The most common high-frequency artifacts include muscle potentials that may contaminate scalp recording and ambient artifact from the mains (power line) current (at 50 or 60 Hz). The mains current-associated artifact should be readily evident, but muscle artifact on scalp EEG is a significant source of misinterpretation and bears further discussion.
Cognition-Associated and Evoked HFOs
Cognitive neuroscientists and psychologists are particularly interested in HFOs that occur in cognitive tests and other evoked responses.27 These normal HFOs have been recorded with both intracranial and scalp EEG. Since it is impractical (and unethical) to perform intracranial recordings without a clinical indication, most of the literature characterizing the intracranially recorded HFOs evoked by motor, sensory, or cognitive stimuli has been obtained from patients undergoing presurgical intracranial EEG monitoring. Examples of normal intracranially recorded HFOs include 80-150 Hz HFOs evoked in the primary auditory and somatosensory cortices by paired auditory and somatosensory stimuli and in the frontal lobe when an element of attention is added to the stimulation paradigm.28 Other normal intracranially recorded HFOs include 40- to 60-Hz oscillations over primary sensorimotor cortices during self-paced motor tasks and 600-Hz, 10- to 15-ms HFOs over the somatosensory cortex evoked by electric median nerve stimulation.29,30 Although these evoked HFO responses are an important window into the role of HFOs in cognitive processing, the results may not be generalizable to patients who do not have refractory epilepsy. Cognitive neuroscientists are actively seeking less invasive techniques for characterizing disorders that do not require intracranial recording. There is a growing literature describing HFOs recorded with scalp EEG during attention and memory tasks, as well as the effects of schizophrenia, dementia, autism, and attention deficit hyperactivity disorder on scalp-recorded HFOs.27 However, the interpretation of scalp EEG data is problematic due to the confounding issue of noncerebral HFOs produced by muscle artifact.
Effect of Muscle on Scalp-EEG-Recorded HFOs
Whitham and colleagues investigated the confounding effect of muscle artifact on scalp-recorded HFOs. They compared subjects in the paralyzed and nonparalyzed states during simultaneous scalp EEG monitoring31,32 and demonstrated that muscle artifact in nonparalyzed patients increased the power of HFOs by 10-100 times the power measured in the paralyzed (muscle-free) state. Although these effects were greatest in electrodes overlying scalp musculature, they were also present in the electrodes around the vertex where scalp muscle artifact should be minimal.31 They further demonstrated that the largest muscle-associated HFOs were induced by tasks that required a motor response or ocular movement.32 The authors succinctly concluded that “it is evident, though perhaps not surprising, that mental activity causes activation of scalp and neck muscles.”31 Should we discard all of the neuropsychiatric data concerning HFOs? It is likely that there is meaningful information to be gleaned from these investigations, particularly in light of intracranial EEG recordings that are free of muscle artifact, but some studies may need to be reevaluated or repeated with alternative study designs. Evoked responses that require a motor response should be viewed with particular caution.
One way to reevaluate these studies may be to compare the spatial and frequency distributions of these HFOs with those elicited by sham tasks that would require similar amounts of ocular movement and/or motor responses. Otsubo et al. described three characteristic differences between intracranially recorded electrocerebral HFOs and those associated with scalp-EEG-recorded ictal and interictal (chewing-associated) muscle artifact.33 First, unlike the intracranially recorded seizures, the muscle-associated HFOs did not trend toward a specific frequency band; rather, the frequency spectrum of muscle-associated HFOs was scattered. Second, the frequency and spatial characteristics of the interictal and ictal muscle artifacts were identical. Third, the intracranial HFOs propagated to adjacent cortex, whereas the muscle-associated HFOs did not exhibit spatial evolution and, instead, remained in one location.33 Investigations using scalp-EEG-recorded HFOs will need to address these characteristics and determine whether their candidate HFOs are likely of cerebral origin before conclusions can be drawn from them.
In epilepsy, the identification and interpretation of HFOs is less fraught with error because much of the data has come from intracranial recording (see Fig. 20.5). The literature regarding intracranially recorded EEG and HFOs can be divided into two broad categories: ictal and interictal. Interictal HFOs consist of “ripples” of fast activity, and ictal HFOs may occur at seizure onset.
Interictal HFOs
Interictal HFOs consist of brief bursts of fast activity that have been termed “ripples.” These brief paroxysms of fast activity were originally discovered by microelectrode depth EEG recordings in rat models of epilepsy and subsequently observed in humans undergoing presurgical evaluations.34,35 Later studies in presurgical patients were able to identify high-frequency bursts using standard macroelectrodes and foramen ovale electrodes.36,37 Investigators have described two distinct types of ripples based upon their characteristic frequency ranges. The line of demarcation has been set at 200 Hz in most studies utilizing microelectrodes and 250 Hz in those using macroelectrodes. Brief oscillations slower than 200-250 Hz (but faster than 80-100 Hz) are known as “ripples,” while those faster than 200-250 Hz (and typically slower than 500 Hz) are called “fast ripples.”34,35,38 Of note, the foramen ovale recordings only characterized ripples of up to 150 Hz.
Ripples likely result from converging IPSPs from rapidly bursting interneurons onto principal neurons or gap junction-mediated synchronization of principal neurons in an epileptogenic network.34,39 Microelectrode recording appears to provide better sampling of fast ripples relative to standard macroelectrode recording, which may be due to the more restricted field of the fast ripples.40 Microrecorded
fast ripples have been attributed to action potentials arising from single neurons (“pure” fast ripples) or to the interference patterns of action potentials arising from slower-firing neurons (“emergent” fast ripples).41 Recent modeling has implicated GABAergic postsynaptic potentials as a source of macroelectrode-recorded fast ripples.42 Regardless of the cellular origins of these waveforms, their clinical relevance is being defined through epilepsy-related investigations.
fast ripples have been attributed to action potentials arising from single neurons (“pure” fast ripples) or to the interference patterns of action potentials arising from slower-firing neurons (“emergent” fast ripples).41 Recent modeling has implicated GABAergic postsynaptic potentials as a source of macroelectrode-recorded fast ripples.42 Regardless of the cellular origins of these waveforms, their clinical relevance is being defined through epilepsy-related investigations.
HFOs occur normally in the rat hippocampus, particularly during exploratory behavior.43 Normal ripple frequency HFOs may occur spontaneously or as evoked potentials in humans in response to auditory or somatosensory stimuli, as described above. Similarly, normal fast ripple HFOs can be evoked by thalamic stimulation in rodents.44 If HFOs are a biomarker of certain disease states, it is important to differentiate between normal and abnormal HFOs. In rats, normal ripples occur bilaterally in the hippocampi, parahippocampal regions, and entorhinal cortex in both epileptic and nonepileptic rats. Fast ripples, on the other hand, are only seen in epileptic rats, and only in structures ipsilateral to seizure onset.
In humans, intracranial recordings in epilepsy patients have recorded HFOs, especially at ripple frequencies, in both normal and abnormal neocortex. When reviewing an intracranially recorded EEG for HFOs, appropriate filtering parameters must be used in order to attenuate the higher-amplitude slower activity. Commonly used criteria define HFOs as consisting of ≥4 oscillations of electrocerebral activity that is >80 Hz and that stands out from the background.45 Normal HFOs have been linked to a number of normal cognitive activities including memory formation and retrieval, in which ripples are more prominently represented than fast ripples, although both may occur.46,47 When pathologic, the majority of fast ripples occur in association with interictal spikes, whereas ripples are seen independently as well as in association with spikes.38,48
Just as interictal spikes and sharp waves vary in frequency with states of arousal, so do ripples and fast ripples. Similarly, with interictal epileptiform activity, both ripples and fast ripples are more likely to occur in non-rapid eye movement (NREM) sleep than in rapid eye movement (REM) sleep or wakefulness.34,38 Both ripples and fast ripples occur simultaneously with interictal spikes, although fast ripples seem more time-linked than ripples.38 Ripples and fast ripples are also more likely to arise from the seizure-onset zone than from other cortical areas, particularly in NREM sleep, and fast ripples in NREM sleep appear to localize the seizure-onset zone most accurately.34,35,38 This is not unexpected, since electric fields generated by rapidly firing IPSPs (ripples) will be generated by a larger volume of cortex than electric fields generated by synchronously firing action potentials (fast ripples).49 Retrospective analysis of epilepsy surgery data has suggested that epilepsy surgery patients are more likely to be seizure-free if HFO-generating cortical regions are resected.50 A prospective investigation, however, found identification of interictal HFOs not to be helpful for individual prognostication although at the group level, there was a positive correlation with seizure-free outcome.51 Interictal HFOs must, therefore, be interpreted within the context of ictal data and should not be utilized in isolation to dictate the extent of resection.
Ictal HFOs
Ictal HFOs are characteristically slower than interictal ripples and fast ripples. As with interictal HFOs, intracranial EEG recordings have provided the best characterization of ictal HFOs. One of the hallmarks of electrographic seizures is evolution in the frequency domain. This evolution is generally from higher frequencies to lower frequencies,52 and understanding the upper limits and localization of those higher frequencies is a work in progress. Due to the above-noted high-frequency filtering effects of the skull and intervening tissues, ictal HFOs are not readily apparent on scalp EEG. Visually obvious, low to moderate voltage paroxysmal fast activity of <30 Hz may be evident at seizure onset. This is a well-defined scalp electrographic accompaniment of both generalized (tonic) and partial seizures.53,54 When attempting to evaluate the higher-frequency range associated with HFOs on scalp EEG, however, one must exercise extraordinary care to avoid misinterpreting subtle (or not-so-subtle) artifactual sources of HFOs. Extrapolating from the work of Whitham and colleagues on scalp-recorded evoked HFOs, we can conclude that analyses of epilepsy-associated HFOs from scalp EEG are suspect, at best.31,32 Techniques to optimize HFO recording, such as sampling from sleep or recording from paralyzed (intubated) patients in the ICU, combined with careful visual exclusion of suspected motor artifacts can increase the likelihood of a meaningful result.
Intracranial recordings, however, mostly avoid muscle artifact and circumvent the filtering effects of the skull. Intracranial recording is not devoid of high-frequency artifact, however, such as that associated with mains current or muscle artifact (reverse breach effect),55 so visual inspection of the recorded data is still necessary. Intracranial recording of extratemporal seizures has demonstrated that seizures characterized by electrodecremental patterns are associated with increased activity in the 40- to 150-Hz range simultaneously with the electrodecrement, particularly in the 80- to 120-Hz range.23 Alarcon et al. suggested that 20-80 Hz localized activity at seizure onset was associated with good surgical outcomes.56 More recent evaluations of surgical outcomes based upon visual inspection of intracranial EEG in neocortical epilepsy have identified the primary determinant of the frequency at onset to be the anatomical location. Temporal neocortical onsets are more likely to be in the beta range vs the gamma range (≥30 Hz) for extratemporal seizures.57 Surgical outcomes were determined more by pathologic substrate than by the frequency components. Park et al. evaluated surgical outcomes in nonlesional neocortical epilepsy and found that gamma or beta range frequencies at ictal onset were more likely to be associated with good outcomes from epilepsy surgery, particularly when the fast activities reproducibly involved the same subsets of
electrodes with each seizure.58 Leung et al. found coexistence of ictal HFOs and cortical excitability (assessed by the cortical afterdischarge to electric stimulation) to be a determinant of seizure-free outcome.59 These investigations show how HFOs are apparent at seizure onset and what role they may play in localizing epileptogenic regions of cortex, but identifying their clinical role will require further research.
electrodes with each seizure.58 Leung et al. found coexistence of ictal HFOs and cortical excitability (assessed by the cortical afterdischarge to electric stimulation) to be a determinant of seizure-free outcome.59 These investigations show how HFOs are apparent at seizure onset and what role they may play in localizing epileptogenic regions of cortex, but identifying their clinical role will require further research.
REVIEW
20.3: Which of the following is NOT true regarding high-frequency EEG?
a. “Ripples” are oscillations of 80-200 Hz related to bursting interneuron-induced IPSPs.
b. “Fast ripples” are 200- to 500-Hz bursts associated with synchronous action potentials.
c. Ripples in the rat hippocampus are associated with exploratory behavior.
d. Fast ripples are seen in epileptic rats associated with interictal spikes.
e. Surgical outcomes are directly related to ictal frequency components.
View Answer
20.3: e. Some studies suggest that inclusion of regions with HFOs in the resection generally improves outcomes, but this did not prove helpful in individual cases, and the relationship remains tentative.
DIGITAL EEG SIGNAL ANALYSIS
Our knowledge of normal and abnormal electrocerebral activity, the optimal means of recording and displaying it, and its clinical relevance was based largely upon visual analysis of EEGs recorded in an analog fashion with ink pens onto paper. With advances in computer technology and increasing memory storage capabilities, digital EEG has largely supplanted analog EEG. We reviewed the basic technology underlying digital EEG in Chapter 2. With the wide availability of digital EEG and digitally stored electrocerebral data, new vistas have opened whereby the data is available not only for visual inspection but also for mathematical manipulation, which could potentially reveal information about the EEG not evident on visual analysis. This mathematical manipulation of digitized EEG data is known as quantitative EEG (QEEG). We will focus here on basic QEEG signal analysis, which many EEG software environments use to help us in routine EEG interpretation.60
Signal Analysis
Signal analysis is the mathematical manipulation of digitally recorded data to analyze and reveal information that is different from the amplitude vs time presentation used for visual inspection of the waveforms. Examples of signal analysis include automated event detection/prediction, monitoring and trending of EEG, source analysis, and frequency analysis.
Automated Event Detection
Automated event detection involves the mathematical comparison of the recorded EEG data with predetermined values. When the recorded signals meet these predetermined criteria, the software flags that portion of the recording for visual inspection. These techniques have been used for spike and seizure detection as well as seizure prediction. Spike and seizure detection software is typically used when large volumes of EEG data (hours to days) must be reviewed and serves to focus the attention of the reviewer on more manageable (and higher yield) aliquots of EEG. The criteria for spike and seizure detection often employ a mathematical re-creation of the process that the reader would ordinarily perform when deciding whether activity is epileptiform. These mathematical simulations of the visual scanning process are approximations at best, so there are no absolute criteria that will fit every kind of epileptiform activity. Criteria can therefore be manipulated to increase or decrease the sensitivity of the detection program. Since the object of the detection software is to evaluate large volumes of EEG and flag suspicious portions for subsequent analysis, the parameters are often set to maximize sensitivity at the expense of specificity. The result is usually a large number of false-positive detections and ideally few false negatives.61 The EEG interpreter can then review the detections more efficiently.
The mathematical analyses used to detect interictal and ictal epileptiform activity are discussed in some detail in Chapter 22. An overview of some of the commonly used methods can be illustrative.
Interictal Epileptiform Activity
Interictal epileptiform activity takes many forms including spikes, sharp waves, and spike- and polyspike-wave discharges (see Chapter 9). The sharpness (determined by the amplitude and frequency characteristics) of these waveforms relative to the surrounding background EEG as well as their spatial distribution serve as the basis for visual detection of interictal epileptiform activity. Most early mathematical recreations of the process of visual analysis have taken one of two approaches. Either they have focused on the frequency, amplitude, and sharpness of the waveforms in question compared with those of the baseline or they have used probabilistic analysis to identify short-duration events that are “improbable.”61 These techniques have approximately an 80%-90% true positive rate.61 False-positive detections can result when there is no spatial component to the analysis. As noted above, when the EEG reviewer assesses a transient, he or she will not only assess the sharpness, amplitude, and frequency of the waveform but also evaluate the topographic field, which is often the primary means of differentiating artifact from epileptiform activity.62 More advanced algorithms have included evaluation of the topography of suspicious transients. Other advanced techniques include the use of neural networks
that can be trained to recognize specific epileptiform activities.63,64 New methods of signal analysis will provide potentially more reliable methods for spike detection.
that can be trained to recognize specific epileptiform activities.63,64 New methods of signal analysis will provide potentially more reliable methods for spike detection.
Ictal Epileptiform Activity
Electrographic seizures are the manifestation of excessively rhythmical electrocerebral activity and can take many forms such as repetitive spike-waves, repetitive polyspikes, low-amplitude desynchronization, and rhythmic waves of a wide variety of frequencies and amplitudes.54 Various repetitive artifacts including eye flutter, movements such as chewing, and EMG can mimic or obscure these patterns on scalp EEG. Detection of electrographic seizure activity is thus inherently more difficult than spike detection. Early seizure detectors concentrated on amplitude criteria to detect the most severe and easily recognizable ictal event, the generalized tonicclonic seizure. These techniques either monitored for the sequence of high-amplitude EEG and EMG activity immediately followed by lower-amplitude suppression65 or filtered the EEG and monitored for the occurrence of high-amplitude, repetitive spikes.66 These systems were useful for the detection of generalized convulsive events, but they were not particularly helpful for the detection of other seizure types. A method for seizure detection widely used since the mid-1980s is based upon the assumption that seizures have increased amplitude, frequency, and/or rhythmicity relative to the background.67,68 This method divides the EEG into 2-second epochs in which the recorded activity is decomposed into “half-waves” whose amplitude and frequency can be compared with the patient’s baseline for a given state of arousal. Activities that had sustained rhythmicity for at least a few seconds with greater amplitude or frequency than the background are detected as seizures.61 This detection method for scalp EEG has a sensitivity of 70%-80% with a 1-3 per hour rate of false-positive detections.69,70 As computational power has increased, topographic characteristics have been incorporated into more advanced seizure detection programs.71 Increasingly sophisticated seizure detection software may allow not only screening large amounts of EEG data but also development of a reliable warning system that can alert patients or caregivers that a seizure is going to occur.
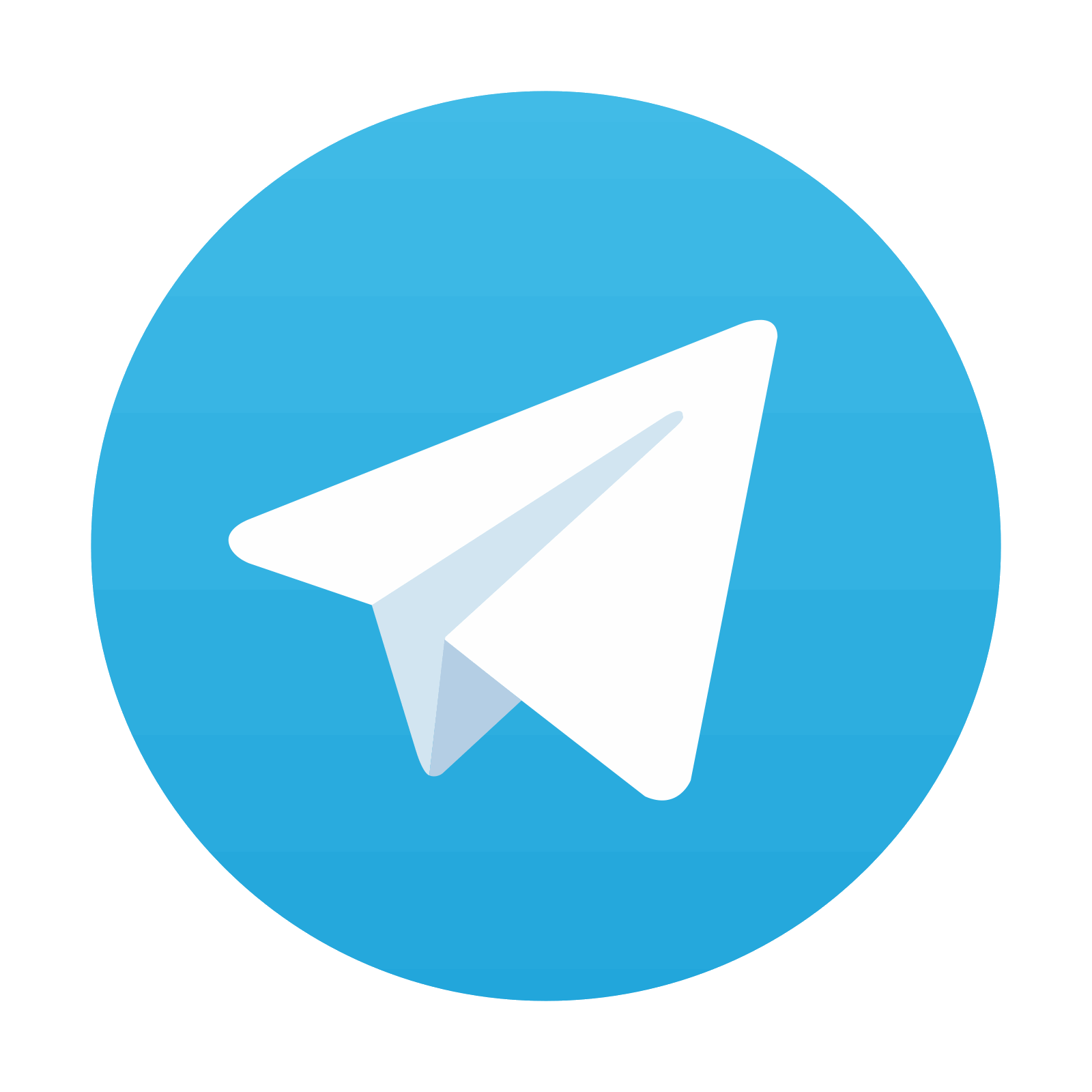
Stay updated, free articles. Join our Telegram channel
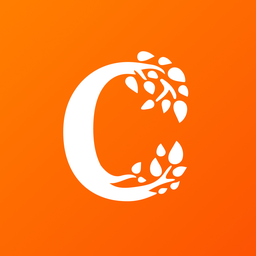
Full access? Get Clinical Tree
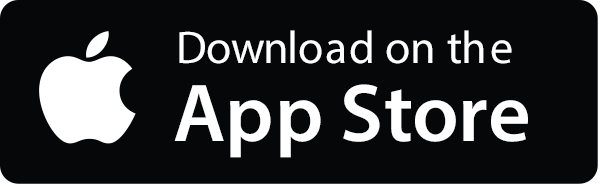
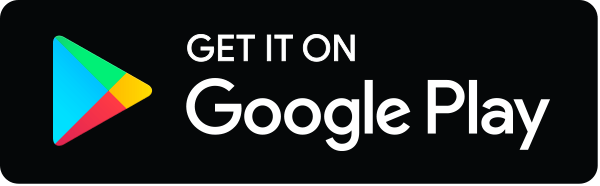
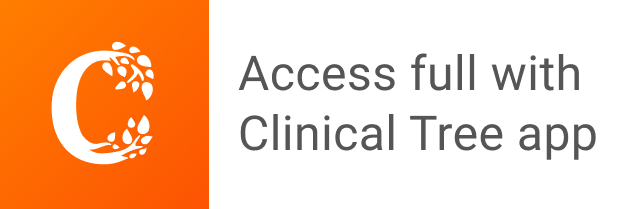