Noninvasive Ventilation in Patients with Respiratory or Neuromuscular Disease
John Seymour
LEARNING OBJECTIVES
On completion of this chapter, the reader should be able to:
1. List the two main types of lung disease and how they impact breathing.
2. Describe the purpose of noninvasive ventilation (NIV) and how it works.
3. Discuss the goals for delivering NIV to a patient with a respiratory or neuromuscular disease.
4. Define modes, settings, and other terms used to implement NIV.
5. Describe the relationship between carbon dioxide levels and ventilation.
6. Discuss the relationship of tidal volume and respiratory rate to alveolar ventilation.
7. Discuss new technologies that can be utilized to track therapy and automatically adjust settings to maintain a desired level of ventilation.
KEY TERMS
Noninvasive ventilation (NIV)
Obstructive sleep apnea (OSA)
Restrictive thoracic disorder
Amyotrophic lateral sclerosis (ALS)
Muscular dystrophy (MD)
Obesity hypoventilation syndrome (OHS)
Chronic obstructive pulmonary disease (COPD)
Continuous positive airway pressure (CPAP)
Inspiration
Expiration
Respiratory rate
Tidal volume
Dead space
Minute ventilation
Alveolar minute ventilation
Hyperventilation
Hypoventilation
Hypocapnia
Hypercapnia
Sleep technologists will see a wide range of patients coming into the sleep laboratory for diagnosis and treatment. These patients will range in complexity from simple obstructive sleep apnea (OSA) patients to complex patients exhibiting periodic breathing. Somewhere in between these extremes of the sleep-disordered breathing spectrum will be those patients with some form of underlying lung disease. Some of these patients will also have the complication of upper airway obstruction.
Respiratory therapists often categorize lung disease into two main types of disease processes. These are restrictive and obstructive disorders. Recognizing the differences in these disease processes will aid the sleep technologist in understanding how noninvasive ventilation (NIV) is used to improve the quality of sleep and life for patients with these diseases.
RESTRICTIVE THORACIC DISORDERS
The term “restrictive thoracic disorder” is used instead of restrictive lung disease because most of the diseases in this category are not really disorders of the lung. These disorders normally involve some problem with the thorax or chest. These patients may have relatively normal healthy lungs but still have a problem breathing. These disorders are characterized by processes that limit the patient’s ability to take a normal-sized breath, something that “restricts” the ability to inspire fully. A few of the more common types of restrictive disorders are discussed in the following paragraphs.
Amyotrophic lateral sclerosis (ALS), also known as Lou Gehrig disease, is an example of a restrictive disorder. This disease is characterized by a gradual destruction of motor neurons in the brain and spinal cord. As these motor neurons are gradually destroyed, the patient loses the ability to move voluntarily, speak, swallow, and breathe. Because this is a progressive disease, it is also an excellent example illustrating the need for monitoring therapy after initiation and then adjusting therapy as needed. These patients will get worse after they are seen in the sleep laboratory and their therapy may need to be adjusted to manage their weakening condition.
Muscular dystrophy is the name for a group of genetically inherited muscle diseases that are characterized by a loss of muscle protein. This loss of muscle protein weakens the muscle to the extent that it can no longer do its assigned task. This is also a progressive disorder like ALS and may lead to inability to perform activities of daily living and could ultimately lead to breathing difficulty as the muscles of breathing become affected.
Obesity hypoventilation syndrome (OHS) is another type of disorder that exhibits restrictive characteristics. Patients with this disorder are obese and exhibit daytime alveolar hypoventilation. These patients do not have chronic obstructive pulmonary disease (COPD) as a cause of their alveolar hypoventilation. Consequences of this daytime alveolar hypoventilation include hypercapnia and hypoxemia. The mechanisms causing the hypoventilation are complex and involve reduced chest wall compliance, impaired respiratory drive, and severe OSA. Treatment of the OSA component with continuous positive airway pressure (CPAP) is beneficial to these patients, but if they continue to have persistent hypercapnia and oxygen desaturation, then bilevel therapy or NIV may have to be utilized (1).
All of these patients with restrictive lung disease have the potential to have difficulty maintaining a normal level of ventilation while they are awake. These patients lose voluntary control of ventilation when sleeping and their breathing can become compromised to the point where serious health consequences can occur.
OBSTRUCTIVE LUNG DISEASE
Unlike most of the restrictive thoracic disorders, obstructive lung disease does involve destructive changes to the lung tissue itself. Obstructive lung disease is more commonly known as COPD. COPD is not the only type of obstructive lung disease, but it is the most common one. COPD typically results from cigarette smoking or in some cases industrial exposure to dust or fumes. Asthma is also a type of obstructive lung disease.
Like OSA, there is an obstruction or airflow limitation occurring in these patients, but COPD is very different from OSA. It differs from OSA in two primary ways. The airflow limitation occurs during the expiratory phase of breathing instead of the inspiratory phase as is the case with OSA. The other way by which COPD differs from OSA is that the obstruction to flow occurs in the distal airways and alveoli of the lung instead of the upper airway. COPD is not diagnosed during a sleep study. It is diagnosed with a pulmonary function test called “spirometry.”
These patients have trouble exhaling the air out of their lungs. In extreme cases, this will cause the patient to inhale before all the air in the previous breath is exhaled. This causes the patient to gradually hyperinflate his or her lungs over time. Eventually, the patient can get to the point where he or she can no longer inhale properly because there is no more room for air in the lungs.
The patient with COPD and OSA is referred to as an “overlap patient” and like the OHS patient will usually benefit from a resolution of OSA symptoms, but may still experience persistent hypercapnia and oxygen desaturations that may need to be treated using bilevel ventilation or NIV. The patient with COPD in need of NIV presents unique challenges to the sleep technologist. Although these patients need additional alveolar ventilation, the sleep technologist needs to be aware that these patients may present with significant airflow limitation, not on inspiration, but on expiration. It is not simply a matter of turning up the respiratory rate or increasing the amount of pressure delivered in order to increase alveolar ventilation. Although it is always important to deliver the properly sized breath to a patient, in COPD patients with hyperinflated lungs, it is just as important to allow the breath to be exhaled.
NONINVASIVE VENTILATION
NIV has been used successfully for decades in acute care environments to assist patients to avoid intubation in cases of acute respiratory failure. The evidence for using NIV for the successful treatment of chronic respiratory failure caused by neuromuscular disease in home care environments is also well documented. The use of NIV to treat chronic respiratory failure due to COPD has been the focus of several recent studies. The practice of placing these COPD patients on minimal levels of NIV is changing. The focus of recent studies into the use of NIV with COPD patients has been on the use of higher pressures, or high-intensity NIV, in order to increase ventilation to the point where carbon dioxide (CO2) reductions in blood gasses are produced (2).
One of the main differences in the use of NIV in the acute care environment and for chronic care at home is the different quality of monitoring and intervention by trained health care professionals. This makes the initial setup and effective titration of NIV in the sleep laboratory all the more critical to the successful treatment of the patient with chronic respiratory failure in the home environment. The patient who has an OSA component in addition to chronic respiratory failure has two strikes against him or her when he or she sleeps. Although eliminating the upper airway component is always helpful, these patients will often require the assistance of NIV to mitigate the impact of their chronic respiratory condition on their sleep, health, and quality of life.
GOALS OF NIV TREATMENT
The goal of NIV is to reduce the work of breathing and to normalize or improve the patient’s blood gasses. It does not matter if the patient has a restrictive or obstructive disorder; the goal is the same. There are some subtle differences in how NIV is applied for these disorders, but the goal remains the same. Complete normalization of blood gasses is not always attained, but reducing the work of breathing is essential and if not attained it is less likely that the patient will be compliant with the therapy. The consequences of a lack of compliance with NIV therapy can be worse than CPAP noncompliance. If the patients don’t use the therapy, there is no benefit.
Anyone who routinely initiates and titrates NIV on patients should experience it for themselves, at least briefly, so they can better relate to what patients feel like. NIV uses pressure support to provide ventilation. Properly adjusted pressure support therapy should follow the patient’s breathing pattern and provide assistance with inspiration and allow for comfortable exhalation. Most patients should be able to control both their respiratory rate and tidal volume if the therapy is titrated properly. Easing the work of breathing for someone is one of the most rewarding things a clinician can do.
BASICS OF NORMAL VENTILATION
The basics of normal ventilation should be reviewed before delving into the details of treatment methods. Knowing what is normal ventilation is important before attempting to bring the patient back toward normal ventilation. Some of the terms the clinician should be familiar with include the following: inspiration, expiration, respiratory rate, tidal volume, dead space, minute ventilation, and alveolar minute ventilation.
INSPIRATION
In normal healthy people, inspiration is achieved when the diaphragm contracts. This causes the diaphragm to flatten out and drop down in the chest cavity. The result is that the amount of space or available volume in the chest cavity increases. This increase in space causes the pressure in the chest to decrease. If the upper airway is not obstructed, then air will move from outside the chest to fill the lungs. The diaphragm is the primary muscle involved in breathing, but the diaphragm can be supplemented by the use of accessory muscles in the chest to help elevate the rib cage upward and outward to further increase the dimensions of the chest cavity, thus creating more negative pressure to draw more air into the lungs.
EXPIRATION
Expiration is normally passive, with the elastic nature of the diaphragm and lungs providing the recoil to push air out of the lungs. Like inspiration, expiration can also be supplemented by the use of accessory muscles if necessary to facilitate expiration. This is most commonly seen in obstructive lung disease where the distal airways collapse and trap air in the lungs. Patients with obstructive lung disease have to work to inhale and to exhale. They have to actively push air out of their lungs in addition to the normal work of breathing to inhale. These patients expend a great deal of energy on just breathing.
RESPIRATORY RATE
The number of times a person breathes each minute is his or her respiratory rate. Respiratory rate can vary from person to person, according to their metabolic needs at the time. Normal respiratory rates are about 10 to 12 breaths per minute.
TIDAL VOLUME
The amount of air that is drawn into the lungs on any single breath is called tidal volume. The size of the tidal volume can vary greatly from person to person on the basis of his or her size and metabolic activity. Normal tidal volumes are in the range of 6 to 8 mL per kg of body weight. Higher tidal volumes delivered by NIV may be uncomfortable, whereas lower tidal volumes are not efficient for achieving alveolar ventilation.
DEAD SPACE
A portion of each inhaled tidal volume does not participate in gas exchange. This portion of the breath is called dead space. There are two types of dead space. Every person has a certain amount of anatomic dead space. This space comprises the larger airways in the lungs and portions of the upper airway. On inspiration, this dead space is the last portion of air from outside of the body that can’t reach the gas exchange areas. On expiration, the anatomic dead space is flushed out and filled with air leaving the alveoli. This air has a high CO2 content and is then pulled back into the alveoli on the next inspiration. The average person has about 150 mL of anatomic dead space (2). Of course, larger people will have larger amount of dead space volumes and smaller people will have smaller dead space volumes.
The other type of dead space is known as “physiologic dead space.” This is air that is inhaled and does
reach the areas where gas exchange is supposed to occur, but for some reason does not fully participate in gas exchange. Proper respiration relies on ventilation matching up with good blood flow to the lungs. Mismatches of ventilation to perfusion can lead to increased amounts of dead space. Physiologic dead space is more of an issue in obstructive lung disorders than it is with restrictive lung disorders. Physiologic dead space is difficult to calculate and in any event it is not always necessary to know what the volume of physiologic dead space is in order to provide adequate therapy.
reach the areas where gas exchange is supposed to occur, but for some reason does not fully participate in gas exchange. Proper respiration relies on ventilation matching up with good blood flow to the lungs. Mismatches of ventilation to perfusion can lead to increased amounts of dead space. Physiologic dead space is more of an issue in obstructive lung disorders than it is with restrictive lung disorders. Physiologic dead space is difficult to calculate and in any event it is not always necessary to know what the volume of physiologic dead space is in order to provide adequate therapy.
MINUTE VENTILATION
A person’s average tidal volume multiplied by his or her respiratory rate determines his or her minute ventilation. Another way to describe minute ventilation is that it is all the air the patient moves in and out of his or her body in a minute.
ALVEOLAR VENTILATION/ALVEOLAR MINUTE VENTILATION
Alveolar ventilation is the effective ventilation. Once dead space is known, then alveolar ventilation is the next calculation that can be made. Alveolar ventilation is calculated simply by taking the tidal volume of the patient and subtracting the dead space from it. This is the alveolar tidal volume. Multiply the alveolar tidal volume by the respiratory rate to determine the alveolar minute ventilation.
RELATIONSHIP OF TIDAL VOLUME TO ALVEOLAR MINUTE VENTILATION
The average amount of anatomic dead space and its relationship to alveolar minute ventilation becomes important when considering assisting a person’s breathing with mechanical ventilation. There are numerous ways to get to an adequate level of alveolar ventilation. Start with delivery of an adequate tidal volume that is comfortable to the patient and then assure that this tidal volume is provided at the correct frequency. That frequency or breath rate should be sufficient to provide the minimum alveolar minute ventilation for that patient. See Figures 50-1 and 50-2 for an illustration of the importance of tidal volume and the impact to alveolar ventilation.
These changes to tidal volume and rate have not changed minute ventilation, but in reality, the alveolar ventilation has been reduced by 10% and a rise in CO2 levels may occur as a result. It can get pretty confusing thinking in terms of alveolar ventilation, dead space, tidal volume, and minute ventilation. The simplest way to approach ventilation is to get the supported breath volume needed correct. Know the patient’s weight and use the guideline of 6 to 8 mL per kg as a starting point for determining tidal volume. The tidal volume calculation is based on body size and there is no need to add any extra volume to account for anatomic dead space (see Table 50-1). Tidal volumes larger than 8 mL per kg should be avoided unless ordered by a physician. Larger tidal volumes may produce effective increases in alveolar ventilation and reductions in CO2, but they do create a risk of discomfort and noncompliance with therapy (3).
CARBON DIOXIDE AND VENTILATION
The common thread between all types of lung disease is that these patients tend to have some degree of hypoventilation. Hypoventilation occurs when a patient either breathes too shallowly or too slowly to remove the CO2 that he or she is producing. The end result is that CO2 levels build up in their bodies, producing something called “hypercapnia,” or elevated CO2 levels.
Table 50-1 Conversion Table for Tidal Volume (Vt) | ||||||||||||||||||||||||||||||||||||||||||||||||
---|---|---|---|---|---|---|---|---|---|---|---|---|---|---|---|---|---|---|---|---|---|---|---|---|---|---|---|---|---|---|---|---|---|---|---|---|---|---|---|---|---|---|---|---|---|---|---|---|
|
Why is CO2 so important? Think of CO2 as the cellular metabolic by-product of life. All of our cells are busy all the time metabolizing or “burning” sugars to produce energy. One of the waste products of this process is CO2. It is released from the cells into the blood-stream and is carried to the lungs where the lungs try to vent this CO2 into the air that enters and leaves the lungs. Thinking of this CO2 as “smoke” from the burning sugar will help in understanding why it is important for it to be removed from the body. When CO2-rich blood reaches the lungs, it is passively transported from the lungs to the air sacs or alveoli. The lungs then need to exchange this CO2-laden air with fresh air from outside the body in order to get rid of the CO2. At a higher rate of ventilation, the CO2 levels in the blood usually are lower. Conversely, if the amount of air being moved through the lungs is reduced, then the CO2 level in the blood usually increases. Another way of stating this is that CO2 levels in the blood are inversely proportional to ventilation. This is one of the most important concepts for fully understanding ventilation.
![]() Figure 50-3 Hypoventilation (low ventilation) is associated with hypercapnia (high CO2), and hyperventilation (high ventilation) is associated with hypocapnia (low CO2). |
The normal range for CO2 is between 35 and 45 mm Hg. Simply put, if the patient’s measured CO2 is above the normal range, then he or she is not getting enough alveolar ventilation or is hypoventilating (see Fig. 50-3). If the measured CO2 is below the normal range, then the patient is getting too much alveolar ventilation or he or she is hyperventilating. Hyperventilation is unusual to see during sleep, except during periods of periodic breathing such as with Cheyne-Stokes respirations. Most of the time hypoventilation/hypercapnia will present clinically.
This brings the discussion full circle back to tidal volumes and the impact they have on alveolar ventilation and CO2 levels. In critical care, it is common to have CO2 levels readily available to the clinician with the use of arterial blood gas (ABG) analysis. Respiratory therapists rely heavily on CO2 measurements to titrate and adjust ventilation. The clinician in the sleep laboratory seldom has access to ABG data. Special lab equipment and qualified clinicians are required to obtain the sample and perform ABG analysis. ABG blood draws are also very painful and would have a negative impact on sleep.
Capnography, or end-tidal CO2 readings, can be used to estimate what the CO2 levels are in the blood, but they are never the same as ABG data. The accuracy of end-tidal CO2 readings obtained during NIV is also impacted by the leak that is a normal component of NIV. Most NIV devices use a leak circuit, just like CPAP, instead of a closed circuit. Capnography may be useful to track how end-tidal CO2 readings are trending, but clinicians should always be cautious when using capnography as a guide for titration during NIV.
Transcutaneous CO2 readings are not impacted by the use of NIV and may be a more reliable measure of what the true CO2 level is in the blood. It can be useful as a trending tool to see if CO2 levels are decreasing as increases in ventilation are made during a NIV titration.
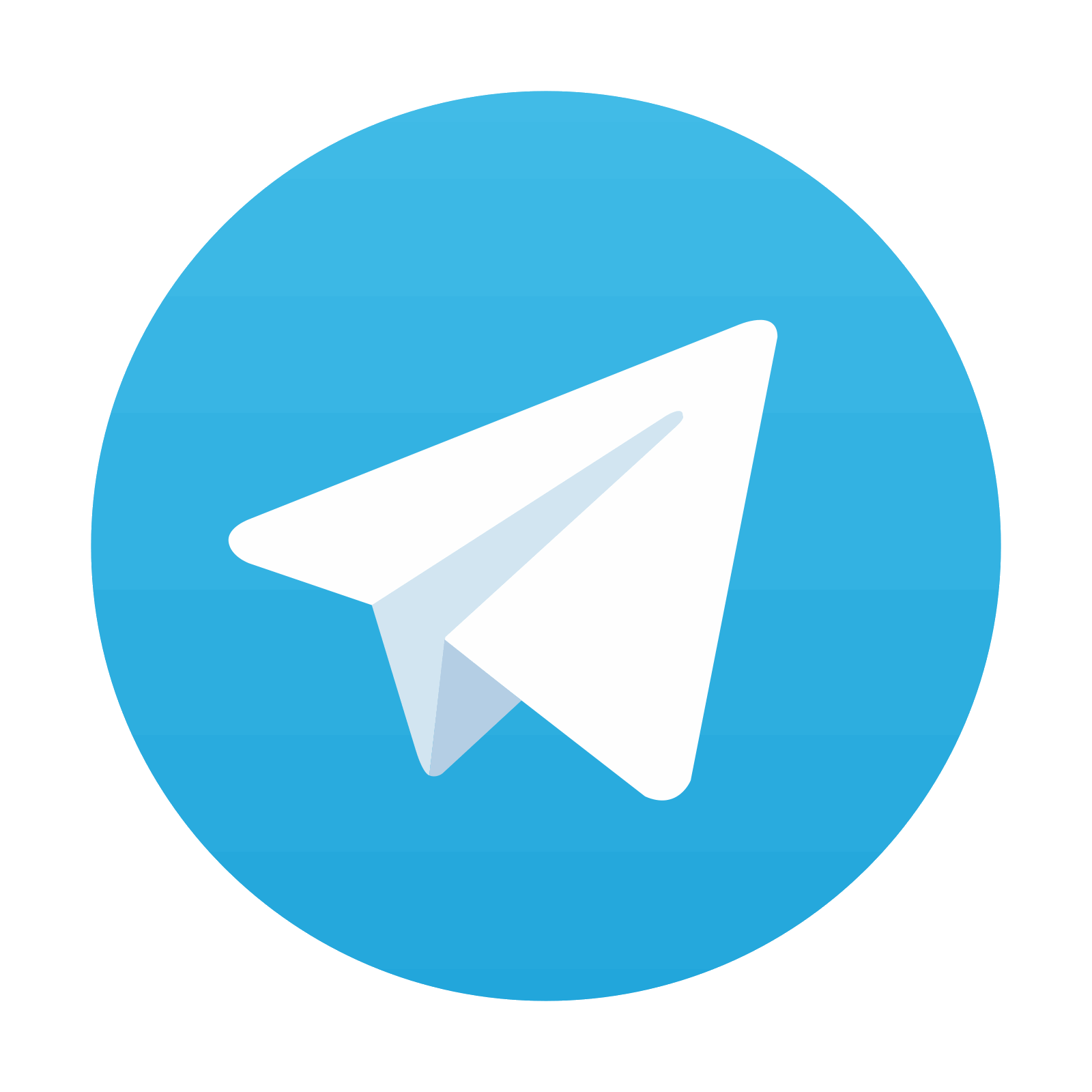
Stay updated, free articles. Join our Telegram channel
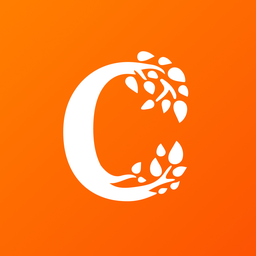
Full access? Get Clinical Tree
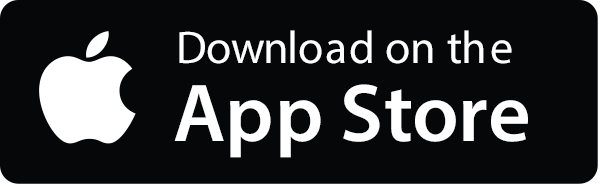
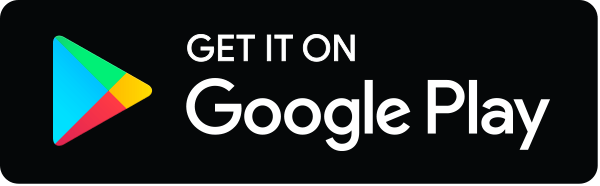