Fig. 13.1
Structural formula of (a) noradrenaline, (b) meta-iodobenzylguanidine (MIBG), (c) guanethidine, and (d) bretylium. MIBG was synthesized on the basis of the structural formulas of guanethidine and bretylium
13.2 The Role of Noradrenaline in Cardiac Sympathetic Nerves
Autonomic control of the heart consists of sympathetic and parasympathetic nerves [1]. The neurotransmitter of the sympathetic system is noradrenaline. The neurotransmitter of the parasympathetic system is acetylcholine. Working together, these systems stimulate or inhibit the heart via adrenergic and muscarinic receptors [1]. Sympathetic output is controlled by regulatory centers in the brain that integrate input signals from other brain sites and receptors throughout the body, such as the carotid sinus and aortic sinus [1]. The heart contains densely distributed postsynaptic sympathetic fibers where noradrenaline functions as a neurotransmitter.
Efferent sympathetic nerves descend in the spinal cord, synapse with preganglionic fibers, synapse with paravertebral stellate ganglia, and innervate the right ventricle and anterior/lateral left ventricle. In the heart, sympathetic nerves follow coronary arteries in the subepicardium, penetrating into the myocardium to regulate cardiac function [1, 2]. Sympathetic nerves consist of a cell body, long axon, and adrenergic postganglionic fibers that branch to form nerve endings. These adrenergic postganglionic fibers control the heart, with the sinoatrial node, atrioventricular node, and atrium receiving the most innervation. The ventricles receive less innervation and Purkinje fibers the least innervation [3]. Sympathetic nerve fibers contain a number of varicose structures, so-called varicosities. The transmission of nerve impulses to the nerve terminal produces nerve terminal depolarization. Synaptic vesicles stored in these varicosities aggregate and integrate into the cell membrane releasing noradrenaline. This is called exocytosis, and noradrenaline is thus released into the synapse between nerve endings and cardiomyocytes, binds to receptors on effector organs, and enhances adenyl cyclase activity through intermediary G proteins [4–6]. Lastly, the impulse is transmitted to cardiac myocytes to modulate physiological functions, such as increasing heart rate and cardiac contraction.
13.3 Kinetics of Noradrenaline
13.3.1 Noradrenaline Synthesis
The synthesis of noradrenaline begins with the synthesis of dopamine. Once dopamine is synthesized from the amino acid tyrosine, which is taken up by active transport from the blood, it is stored in synaptic vesicles (Fig. 13.2). The enzyme dopamine beta-hydroxylase further hydroxylates dopamine into noradrenaline. Tyrosine is converted to levodopa via the enzyme tyrosine hydroxylase and converted to dopamine by DOPA decarboxylase. The reaction catalyzed by tyrosine hydroxylase is the rate-limiting step in the production of catecholamines. The end product, noradrenaline itself, controls noradrenaline synthesis by feedback to the rate-limiting enzyme, and the released noradrenaline promotes increased tyrosine hydroxylase activity. Dopamine beta-hydroxylase, which catalyzes the conversion of noradrenaline from dopamine, is localized in tissues that synthesize catecholamines, such as noradrenergic neurons and chromaffin cells, and is confined within vesicles [3].


Fig. 13.2
Kinetics of noradrenaline and MIBG in cardiac sympathetic nerve ending (Reprinted and adapted with permission from FUJIFILM RI Pharma Co., Ltd.)
13.3.2 Noradrenaline Release, Uptake, and Inactivation
Relatively little noradrenaline released from nerve endings binds to receptors, and most of the remaining noradrenaline (80–95 %) undergoes active reuptake into nerve endings (uptake-1 mechanism). This mechanism is facilitated by the specific noradrenaline transporter (NAT, also known as the norepinephrine transporter, or NET) protein [7]. This uptake mechanism, via NET, is a “specific” sodium-dependent process characterized by high affinity, low capacity, saturability, and temperature and ouabain sensitivity [8]. It is an important inactivation mechanism to inhibit excessive noradrenaline increments in the interstitial myocardium.
Noradrenaline taken into nerve endings is inactivated by metabolism, but this process is limited, and most noradrenaline is repackaged into noradrenaline-storing vesicles by another active transport process, facilitated by the specific vesicular monoamine transporter (vMAT) protein, and reused in exocytosis [7]. On the other hand, noradrenaline not binding to receptors or undergoing reuptake is taken up by nonneuronal postsynaptic cells, probably by sodium-independent passive diffusion. Such “nonspecific” low-level, energy-independent, unsaturable, extraneuronal uptake takes place in all cells and is sometimes called uptake-2 [7, 9, 10]. Under ideal conditions in vitro, the “specific” uptake process is ~50 times more efficient than passive uptake [11]. The remaining noradrenaline spills over into the blood. Finally, metabolic enzymes deactivate noradrenaline. If it does not undergo reuptake or is not taken up by passive diffusion, it is catabolized by catechol-O-methyltransferase (COMT) outside nerve cells. If it undergoes reuptake, but is not repackaged in noradrenaline-storing vesicles, it is catabolized by monoamine oxidase (MAO). The final product of these pathways is vanillylmandelic acid.
13.4 History of the Development of MIBG
13.4.1 Cardiac Imaging Before MIBG
MIBG, an analog of guanethidine (an adrenergic neuron-blocking agent), is a radioactive tracer of noradrenaline and used to evaluate presynaptic sites of the adrenergic system. It is virtually the only radiotracer used for scintigraphy studies of the cardiac autonomic nervous system [7]. The history of its development dates to the 1970s. At the time, noradrenaline labeled with 3H showed rapid localization to the heart. Myocardial imaging with noradrenaline was used often, and noradrenaline labeled with the positron-emitting nuclide 11C was developed. However, this method required a hospital cyclotron and a positron emission tomography (PET) device and thus was not suitable for implementation in conventional facilities. Cardiac tracer development was an unintended result of efforts by Dr. William H. Beierwaltes, then Chief of Nuclear Medicine at the University of Michigan, to develop scintigraphic imaging for adrenal diseases, particularly neoplasms associated with the medulla [1, 12]. It is impossible to fully appreciate the development of MIBG as a cardiac radiotracer without discussing the events leading to its conception as a noninvasive imaging tool for clinical endocrinology [12]. Initially, their group was developing scintigraphic imaging for adrenal disease. They were exploring iodine (I)-labeled analogs of the adrenergic-blocking antiarrhythmic drug bretylium (Fig. 13.1d) and found that the ortho-iodo-bretylium analog was effectively taken up by the heart [13]. However, in later research, they found that the para-iodo-bretylium analog effectively imaged the adrenal medulla and concluded that the para-iodo-bretylium analog was better suited for portraying the adrenal medulla [14].
13.4.2 Usability of MIBG
The efforts of Dr. Beierwaltes were continued by Dr. Donald M. Wieland. In 1978, he synthesized five 125I-labeled bretylium analogs, including para– and ortho-iodo-bretylium analogs. Biodistribution studies demonstrated that the ortho-iodo-bretylium analog had higher uptake levels in the adrenal medulla than para-iodo compounds, which was different from the results of previous studies. In the summer of 1978, when reviewing adrenergic neuron-blocking agents such as guanethidine, he found an article on the adrenergic neuron-blocking activity of benzylguanidines written by Short and Darby [15]. Struck by the structural similarity between benzylguanidines and the iodo-bretylium he had recently worked on, he decided to synthesize and study 125I-ortho-iodobenzylguanidine (OIBG) and 125I-para-iodobenzylguanidine (PIBG) as potential adrenal medulla imaging agents [12] (Fig. 13.3). In January 1979, the first biodistribution studies of 125I-labeled OIBG and PIBG were performed in dogs. The results showed that the ortho-substituted analog OIBG had significantly lower uptake in the adrenal medulla than para-substituted PIBG, in contrast to the previous findings with iodo-bretylium analogs [12]. Since the results with the ortho– and para-iodobenzylguanidines were opposite from what had been observed with the iodo-bretyliums, he felt that a thorough assessment of the structure-activity relationships for iodobenzylguanidines was necessary. He immediately began synthesizing the third possible ring-iodinated structure, MIBG [12]. In 1979, biodistribution studies of MIBG were performed and showed uptake levels comparable with PIBG. After a detailed evaluation, it was concluded that although PIBG had a somewhat higher uptake in the adrenal medulla, MIBG was more metabolically stable, undergoing less deiodination in vivo. Thus MIBG proved to be the optimal radioiodinated benzylguanidine for adrenomedullary imaging [16]. He also performed research on three isomers (para, ortho, meta) and found high early accumulation in the heart [17]. In these studies, the para– and meta-isomers showed good accumulation in the adrenal medulla and had high affinity for sympathetic nerves. However, thyroid uptake was significantly increased with para– and ortho-isomers, while thyroid and liver uptake was low with the meta-isomer. Thus the meta-isomer was considered to be the optimal radioiodinated benzylguanidine for adrenomedullary and cardiac imaging. Wieland et al. studied the biodistribution in animals, such as dogs, and found that MIBG was useful as an adrenomedullary and cardiac-imaging agent [17, 18]. In 1981, he succeeded in performing cardiac imaging of dogs and monkeys using 131I and 123I-MIBG [19].


Fig. 13.3
Structural formula of (a) ortho-iodobenzylguanidine (OIBG) and (b) para-iodobenzylguanidine (PIBG)
13.4.3 Human and Clinical Application of MIBG
The first cardiac-imaging assessment in humans was a study of five normal male volunteers conducted by Kline et al. in 1981 [20]. They administered MIBG intravenously and reported that the left ventricular myocardium could be visualized after 1–2 min [20]. They also concluded that MIBG is a new agent for human myocardial imaging that may provide quantitative information on myocardial catecholamine content, and with its 123I label, this radiopharmaceutical can be used without special equipment in a typical nuclear medicine laboratory [20]. When first developed, MIBG was labeled with 131I and used to detect various tumors [9]. In the United States, only 131I-MIBG has been clinically approved for neuroendocrine tumor imaging and occasionally for therapy of these tumors [21]. However, 123I-MIBG was preferred because 131I gives off relatively high-energy (365 keV) gamma emissions, emits beta particles, and has a relatively long half-life (approximately 8.02 days). 123I emits predominantly gamma photons, with energies of 159 keV, and has a half-life of 13.2 h [9, 21]. It is easily imaged and well tolerated. Later, MIBG imaging was speculated to be useful in evaluating heart failure and identifying patients with autonomic neuropathies predisposed to arrhythmias and sudden cardiac death [22–24]. However, the efforts at Michigan shifted toward PET compounds, resulting in clinical MIBG studies being performed mostly in Europe and Japan [1].
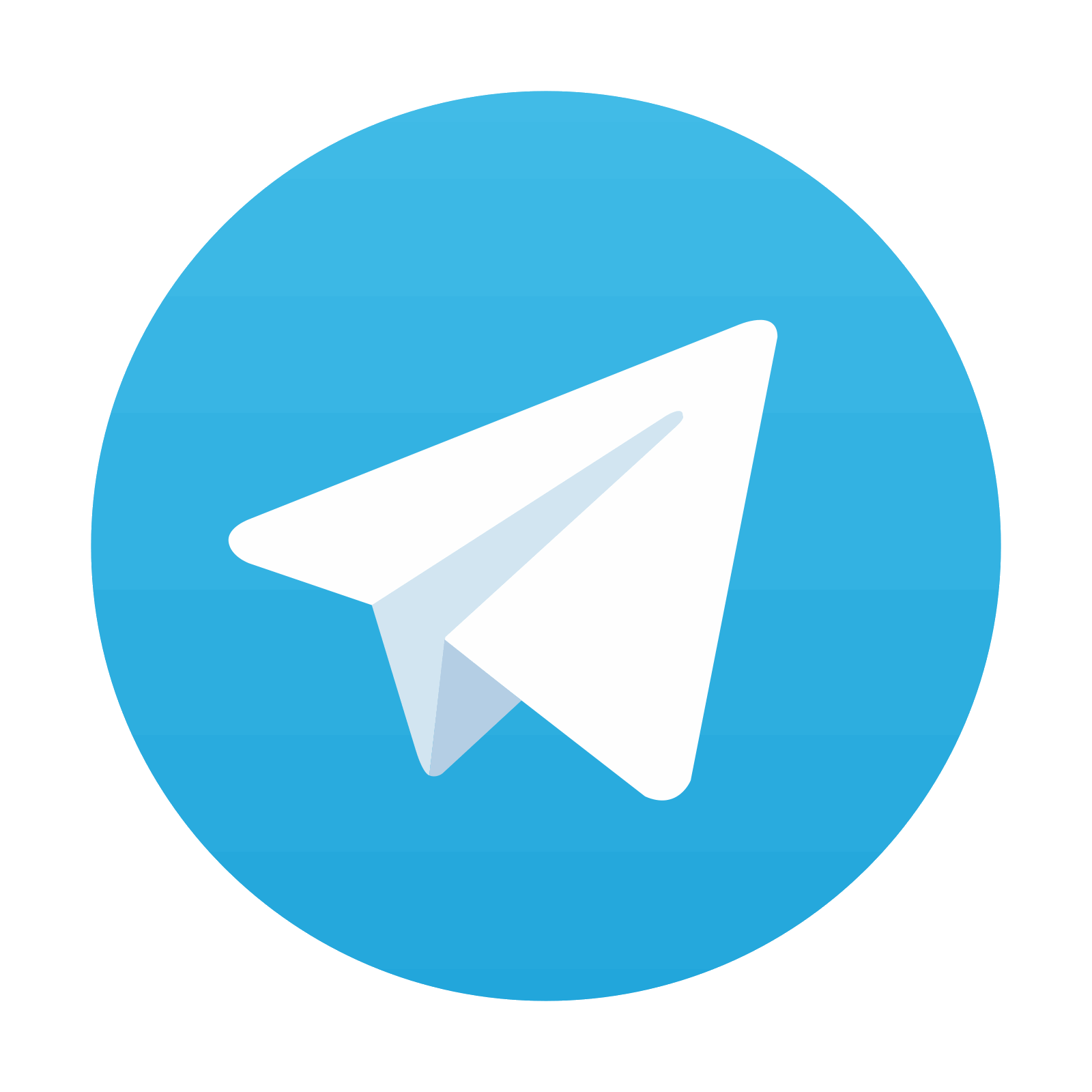
Stay updated, free articles. Join our Telegram channel
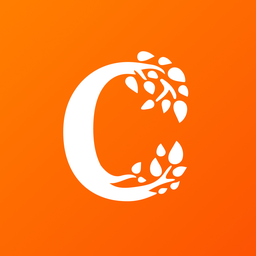
Full access? Get Clinical Tree
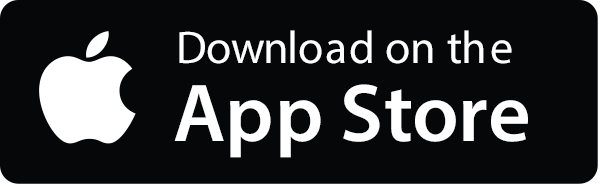
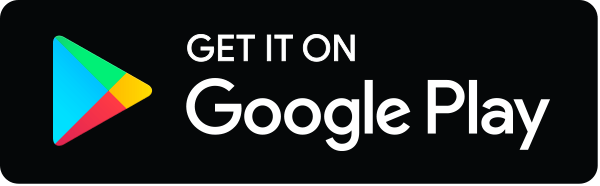