Chapter 4 4.1 Sleep in Animals and the Phylogeny of Sleep The amount of sleep required by animals varies greatly. The horse sleeps less than 2 hours a day, and the big brown bat sleeps 20 hours a day. Rapid eye movement (REM) sleep amounts show even greater variation across species (Fig. 4.1-1). The platypus has the most REM sleep of any animal, approximately 8 hours a day (Video 4.1 and Fig. 4.1-2). Dolphins and other cetaceans do not appear to have any REM sleep, birds have very little, and conclusive evidence for the presence of REM sleep in reptiles is lacking. Figure 4.1-1 Sleep time in mammals. Figure 4.1-2 Brainstem rapid eye movement (REM) sleep state in the platypus. Recent findings undermine the idea that sleep has a vital universal neural or physiologic function across species. Dolphins and other cetaceans never have high-voltage slow waves in both sides of the brain, in contrast to all terrestrial mammals (Fig. 4.1-3). Instead, they have unihemispheric slow waves with closure of the eye contralateral to the hemisphere with slow waves. Furthermore, killer whale and dolphin mothers and their calves are continuously active, and calves keep both eyes open for 2 or more months after birth. No rebound of inactive behavior follows. During this period, the calf’s brain and body grow to their prodigious size and capacity without any apparent need for sleep. An equally remarkable observation has been made that adult dolphins working for reward can discriminate between visual stimuli presented at 30-second intervals on their left or right sides, 24 hours a day, for as long as 15 days. During this time their performance shows no progressive decline, and no rebound of inactivity follows the continuous vigilance task. In contrast, humans whose sleep is interrupted on a similar schedule are dramatically impaired, demonstrating the variability of sleep regulation across species. Figure 4.1-3 Cetacean sleep: unihemispheric slow waves in cetaceans. Numerous attempts have been made to correlate the variation in sleep time across species with physiologic variables such as body mass, life span, brain size, brain/body weight ratio, and litter size. However, such studies have not identified correlations that account for a significant amount of the cross-species variance (Fig. 4.1-4 and Fig. 4.1-5). The few weak and inconsistent correlations that have been reported appear to be largely a function of the way the data are handled and which animals are excluded from the dataset. The definitive study of the phylogeny of REM and non-REM (NREM) sleep times in birds concluded that none of the physiologic parameters typically studied in mammals showed even a weak relationship with sleep time. However, one relation appears to be consistent across both birds and mammals: species that eat food with low caloric density, such as herbivores, sleep significantly less than those that eat more nutritionally dense foods (carnivores; see Fig. 4.1-5). In other words, if a species needs to eat more than 12 hours a day, it cannot sleep more than 12 hours; animals have evolved to adjust sleep time appropriately to such waking needs. Most species, including humans, can reduce sleep to acquire food, avoid predation, mate, be available to their young during critical periods, and to deal with other needs. Figure 4.1-4 Sleep amount is not proportional to the relative size of the cerebral cortex or to the degree of encephalization, as illustrated by a comparison between the bushtail possum and the elephant. Figure 4.1-5 Sleep, brain, and life span parameters across mammalian orders and the avian class. Jones, SG, Paletz, EM, Obermeyer, WH, Hannan, CT, Benca, RM. Seasonal influences on sleep and executive function in the migratory white-crowned sparrow (Zonotrichia leucophrys gambelii). BMC Neurosci. 2010; 11:87. Kripke, DF, Garfinkel, L, Wingard, DL, Klauber, MR, Marler, MR. Mortality associated with sleep duration and insomnia. Arch Gen Psychiatry. 2002; 59:131–136. Lesku, JA, Rattenborg, NC, Valcu, M, et al. Adaptive sleep loss in polygynous pectoral sandpipers. Science. 2012; 337:1654–1658. Lyamin, O, Pryaslova, J, Lance, V, Siegel, J. Animal behaviour: continuous activity in cetaceans after birth. Nature. 2005; 435:1177. Ridgway, S, Keogh, M, Carder, D, et al. Dolphins maintain cognitive performance during 72 to 120 hours of continuous auditory vigilance. J Exp Biol. 2009; 212:1519–1527. Roth, TC, Lesku, JA, Amlaner, CJ, Lima, SL. A phylogenetic analysis of the correlates of sleep in birds. J Sleep Res. 2006; 15:395–402. Siegel, JM. Clues to the functions of mammalian sleep. Nature. 2005; 437:1264–1271. Siegel, JM. Do all animals sleep? Trends Neurosci. 2008; 31:208–213. Siegel, JM. Sleep viewed as a state of adaptive inactivity. Nat Rev Neurosci. 2009; 10:747–753. 4.2 Normal Sleep in Humans All organisms have periods of activity and inactivity. This is true from viruses to the most complex of mammals. Such a pervasive finding suggests that sleep is basic to life (Box 4.2-1). This chapter reviews normal sleep, the function and neurophysiology of the evolution of sleep, and the ontogeny of sleep. To sustain life, no more than 3 to 5 hours of sleep a night are required. However, this amount of sleep leads to sleepiness, impaired performance, and reduced executive functions. To sustain optimal alertness throughout the day, the requirement varies across individuals, with the mean being 7 to 8 hours for adult humans (Table 4.2-1). The presumed function of rapid eye movement (REM), non-REM (NREM), and awake states is provided in Figure 4.2-1. Figure 4.2-2 describes the neurotransmitters involved in wakefulness-generating neural networks, which include glutamate, acetylcholine, norepinephrine, dopamine, histamine, hypocretin, and serotonin, and provides the function and location of each. The interaction of the neuromediators of sleep on brainstem structures responsible for arousal and sleep is described in Figures 4.2-3 and 4.2-4. Figure 4.2-1 The function of the two types of sleep. Figure 4.2-2 Neurochemistry of sleep and wakefulness. Figure 4.2-3 Brainstem-mediated arousal and sleep induction. Figure 4.2-4 The brain in the awake state illustrates important arousal and sleep centers and pathways of neurotransmission. Wakefulness is promoted by the ascending brain arousal systems; inhibition of these systems promotes sleep (Fig. 4.2-5). The brainstem reticular activating system (RAS) contains neurons that inhibit sleep-generating neurons and activate cortical neurons through ascending projections via the thalamus, hypothalamus, and basal forebrain. Descending projections from the reticular formation to the spinal cord are important for maintaining postural control and muscle tone. Figure 4.2-5 Hypothalamic mechanisms in sleep-wake control. Key physiologic changes that occur during the transition from wakefulness into sleep are shown in Figures 4.2-6 through 4.2-8. Figure 4.2-6 In mammals, the two types of sleep are rapid eye movement (REM) and non-REM (NREM).
Normal Sleep
Carnivores are shown in dark red, herbivores in green, and omnivores in blue. Sleep times in each of these differ significantly, but carnivores sleep significantly more than herbivores. Sleep amount is an inverse function of body mass over all terrestrial mammals (black line). This function accounts for approximately 25% of the interspecies variance (bottom right) in reported sleep amounts. Herbivores are responsible for this relation because body mass and sleep time were significantly and inversely correlated in herbivores but were not correlated in carnivores or omnivores. (From Siegel JM: Clues to the functions of mammalian sleep. Nature 2005;437:1264–1271.)
REM and twitches can occur while the forebrain is showing a slow-wave activity pattern. Electroencephalogram (EEG), electrooculogram (EOG), electromyogram (EMG), and EEG power spectra of samples show sleep-wake states in the platypus. (From Siegel JM, Manger PR, Nienhuis R, et al: Sleep and the platypus. Neuroscience 1999;91:391–400.)
Top, Immature beluga, adult dolphin, and section of adult dolphin brain. Electroencephalographic (EEG) readings of adult cetaceans, represented here by the beluga, are shown during sleep. All species of cetacean so far recorded have unihemispheric slow-wave activity. Top traces show left and right EEG activity. The spectral plots show 1 to 3 Hz power in the two hemispheres over a 12-hour period. The pattern in the cetaceans contrasts with the bilateral pattern of slow waves seen under normal conditions in all terrestrial mammals, represented here by the rat (bottom traces). (From Siegel JM: Clues to the functions of mammalian sleep. Nature 2005;437:1264–1271.)
REM, rapid eye movement [sleep]. (From Siegel JM: Clues to the functions of mammalian sleep. Nature 2005;437:1264–1271.)
The strongest correlate of sleep time across species is diet. Animals that eat food with high caloric density do not need to spend as much time ingesting food as animals that eat food with low caloric density. In zoos and laboratories, where most sleep studies have been done and animals are well fed, carnivores sleep more than omnivores, who sleep more than herbivores. However, food deprivation increases waking and decreases sleep in carnivores and omnivores. Flexibility in sleep time increases the likelihood that energy input and output will be equalized and that there will be time for other essential tasks, such as mating and care of young. +++ carnivores; ++ omnivores; + herbivores. Sleep durations in hours/24-hour period; life span in years. (Owl data from http://Birdsflight.com; degu data from http://www.Degus-international.org; greater short-nosed fruit bat data from http://designeranimals.wikispaces.com; photos of other animals courtesy of Wikipedia Commons site.)
How Much Sleep is Enough?
Neurophysiology of Sleep and Wakefulness
Our evolving understanding of the function of rapid eye movement (REM) and non-REM (NREM) sleep and why we need both is based on some interesting hypotheses. First, NREM sleep may allow brain cells a chance to undergo repair. Second, interrupted release of monoamines during REM sleep may allow the brain’s receptors for these neurotransmitters to recover and regain full sensitivity, which may help with regulation of learning and mood. Third, the potent neuronal activity of REM sleep in early life may allow the brain to develop properly. (Modified from Joel M. Siegel: Why we sleep: the reasons that we sleep are gradually becoming less enigmatic. Scientific American, November 2003. Accessed online at http://www.scientificamerican.com/article.cfm?id=why-we-sleep.)
The key neurons responsible for sleep induction and arousal.
The reticular activating system (RAS) oversees the wakefulness state through activity of four subnuclei: (1) the locus ceruleus (LC), which releases norepinephrine (NE); (2) the dorsal raphe nuclei (DRN), which release serotonin (5-HT); (3) the pedunculopontine (PPT) nuclei; and (4) the laterodorsal tegmentum (LDT), which is associated with acetylcholine (Ach). The PPT-LDT neurons are active in wakefulness sa well as REM sleep and are believed to be the REM “on” switch. Lesions in the PPT-LDT area do not affect wakefulness but lead to loss of REM sleep. Slow-wave sleep (SWS) is modulated through the LC and DRN by decreasing the release of NE. Wakefulness is achieved through the combined activity of the PPT, LC, and DRN nuclei releasing Ach, NE, and 5-HT, respectively. The primary neurotransmitter of REM is Ach. SWS is achieved through suppression of the wakefulness neurotransmitters NE and 5-HT and release of Ach. GLY, glycine. (Modified from Webster HH, Jones BE: Neurotoxic lesions of the dorsolateral pontomesencephalic tegmentum-cholinergic cell area in the cat. II. Effects upon sleep-waking states. Brain Res 1988; 458[2]:285–302.)
A, Cholinergic input (orange) from the laterodorsal tegmental (LDT) and pedunculopontine (PPT) nuclei project through the thalamus and facilitate thalamocortical transmission of arousal signals. A second pathway projects through the hypothalamus to cortical centers and facilitates the processing of thalamocortical inputs that arise from midbrain centers, including the noradrenergic (blue) locus coeruleus (LC); the serotonergic (purple) dorsal raphe (Raphe); the histaminergic (pink) tuberomammillary nucleus (TMN); and the dopaminergic (yellow) ventral periaqueductal grey matter (VPAG). This pathway also receives input from the cholinergic (orange) basal forebrain (BF) and the peptidergic neurons of the lateral hypothalamus (LH) and perifornical neurons (PeF), which contain hypocretin or melanin-concentrating hormone (light green). The melatonergic (red) neural network affects arousal and sleep through regulation of circadian rhythms. This internal biologic clock originates in the suprachiasmatic nucleus (SCN), projects through the dorsomedial hypothalamus (DMH), and sends inhibitory signals to the γ-aminobutyric acid (GABA)-ergic (grey) ventrolateral preoptic nucleus (VLPO) of the hypothalamus. B, Artistic rendering of the human brain in the sleeping state illustrates important sleep and arousal centers and pathways of neurotransmission. The VLPO of the hypothalamus sends descending GABAergic inhibitory signals to the midbrain arousal centers that include the PeF, TMN, VPAG, raphe, LDT and PPT, and LC. During the early hours of dark periods, the pineal gland (Pin) releases melatonin (red), which has inhibitory effects on the SCN and DMH of the melatonergic system. Nuclei that control neural activity during REM sleep have been identified in the pontine midbrain. The pericoeruleus (PC) and parabranchial (PB) nuclei send glutaminergic (green) projections through the BF to affect cortical activity during REM sleep, and projections from the sublaterodorsal nucleus (SLD) send glutamatergic signals through the spinal cord to induce the atonia characteristic of REM sleep. (From Wafford KA, Bjarke E: Emerging anti-insomnia drugs: tackling sleeplessness and the quality of wake time. Nat Rev Drug Disc 2008;7:530–540.)
Wakefulness
Importance of wakefulness- and sleep-promoting systems in the hypothalamus has been recently emphasized. These systems innervate the ascending arousal systems (see Fig. 4.2-1) and excite and inhibit the system, respectively, to mediate the effects. A, Hypocretin neurons in the lateral hypothalamic area innervate all the ascending arousal systems as well as the cerebral cortex. B, Neurons of the ventrolateral preoptic (VLPO) area produce γ-aminobutyric acid and galanin, which inhibit all the arousal systems during non–rapid eye movement sleep. BF, basal forebrain; LC, locus coeruleus; LDT, laterodorsal tegmental nuclei; PPT, pedunculopontine; SN, substantia nigra; TMN, tuberomammillary nucleus; VTA, ventral tegmental area. (From Basic sleep concepts, science, deprivation, and mechanisms of neurotransmitters and neuropharmacology of sleep/wake regulations. In Nishino S [ed]: Encyclopedia of sleep. Palo Alto, CA, 2013, Stanford University School of Medicine, pp 395–406. Accessed online at http://dx.doi.org/10.1016/B978-0-12-378610-4.00087-5.)
Transition Between Wakefulness and Sleep
These sleep types are defined in terms of electrophysiologic signs detected with a combination of electroencephalography (EEG), electrooculography (EOG), and electromyography (EMG), the measurement of which in humans is collectively termed polysomnography. REM sleep—also known as paradoxical, active, or desynchronized sleep—is characterized by awakelike and “activated” (high-frequency, low-amplitude, or “desynchronized”) signals in the EEG; singlets and clusters of REMs in the EOG; and very low muscle tone (atonia) in the EMG. Note that the term desynchronized for the activated states of waking and REM has been rendered obsolete by the discovery of highly synchronized γ-frequency (30 to 80 Hz) activity in these states. NREM sleep is divided into four stages that correspond to increasing depth of sleep, as indicated by progressive dominance of the EEG by high-voltage, low-frequency “synchronized” wave activity. Such low-frequency waves dominate the deepest stages of NREM (stages 3 and 4, also termed slow-wave sleep). Stage 2 NREM is characterized by distinctive sleep spindles, K-complex waveforms, and a slow (<1 Hz) oscillation, which influences their timing. A shows the characteristic waveforms of the different sleep stages. Changes in peripheral physiology associated with the sleep stages is shown in C. NREM and REM sleep alternate in each of the four or five cycles that occur in each night of adult human sleep. Early in the night, NREM sleep is deeper and occupies a disproportionately large amount of time, especially in the first cycle, when the REM epoch may be short or aborted. Later in the night, NREM sleep is shallow, and more of each cycle is devoted to REM (red bars). B illustrates these changes over the course of a night’s sleep. A depicts, in detail, features of an early-night sleep cycle in which NREM reaches its greatest depth at stages 3 and 4 (delta-sleep), whereas C depicts a late-night cycle in which NREM descends only to stage 3. The constant period length of the NREM-REM cycle indicates that it is timed by a reliable oscillator, the amplitude of which varies according to extrinsic factors. The cyclic organization of sleep varies within and among species. The period length of each REM-NREM epoch increases with brain size across species, and the depth and proportion of the NREM phase in each cycle increases with brain maturation within species. NREM sleep complexity is a function of brain systems, such as the thalamocortical circuitry, that reach their maximum development in mature humans only to decline in postmature age. It can therefore be concluded that the differentiation of sleep is a function of brain differentiation, a rule that indicates both mechanistic and functional links between sleep and other brain functions. (From Pace-Schott EF, Hobson JA: The neurobiology of sleep: genetics, cellular physiology and subcortical networks. Nat Rev Neurosci 2002;3:591–605.)Stay updated, free articles. Join our Telegram channel
Full access? Get Clinical Tree
Normal Sleep
Only gold members can continue reading. Log In or Register a > to continue
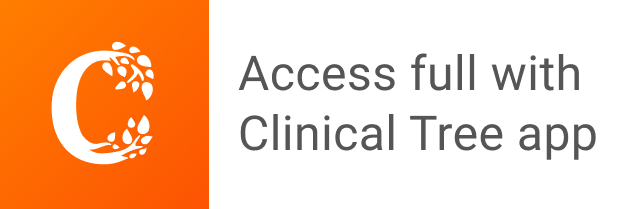