2 Normal Spinal Cord Development and the Embryogenesis of Spinal Cord Tethering Malformations Mark S. Dias and Elias B. Rizk Understanding normal and abnormal embryology of the nervous system is vital for the neurosurgeon treating patients with dysraphic malformations, and not just as an academic exercise. Such understanding not only allows an appreciation for how (and when) these malformations might arise during embryogenesis, it also improves the surgeon’s understanding of the anatomical relationships between the malformation and spinal cord during repair and/or tethered cord release. This chapter briefly reviews several of the earlier embryological events in the formation of the spinal cord, as well as the various ways in which development might go awry. The chapter provides a detailed review of the principles underlying normal early neural development and applies these principles to the embryogenesis of dysraphic malformations. During the first 4 days after fertilization postovulatory day (POD) 1 to 4, the human embryo undergoes approximately five cell divisions to form a mass of ∼32 cells (the blastocyst), which surrounds a central cavity (the blastocystic cavity). The blastocyst contains an eccentrically located inner cell mass (ICM), the embryonic cell proper, and a thinner surrounding ring of cells, the trophoblast (Fig. 2.1). By POD 4, the ICM develops two distinct layers: cells on the dorsal surface, adjacent to the trophoblast, form the epiblast, whereas cells on the ventral surface, adjacent to the blastocystic cavity, form the hypoblast.1 By POD 7 to 12 two additional cavities develop (Fig. 2.1): the amnionic cavity appears between the epiblast and the overlying trophoblast cells, while the umbilical vesicle (or yolk sac) appears below the hypoblast. The epiblast is therefore adjacent to the amnionic cavity, and the hypoblast is adjacent to the umbilical vesicle. By POD 13, the hypoblast thickens cranially; this portion of the hypoblast is the prochordal plate. The prochordal plate will eventually give rise to the cephalic mesenchyme and to portions of the foregut.1 During the second embryonic week gastrulation transforms the embryo from a two-layered structure containing epiblast and hypoblast into a three-layered structure containing ectoderm, mesoderm, and endoderm. A midline structure, the primitive streak, first develops at the caudal end of the blastocyst at POD 13 and elongates cranially over the next 3 days. It reaches its full length by POD 16 and then begins to regress back toward the caudal pole of the embryo. The primitive streak is contiguous cranially with the primitive knot, or Hensen node; in the middle of Hensen node is a small indentation, the primitive pit. Along the length of the primitive streak is located a midline trough, the primitive groove, which is contiguous cranially with the primitive pit.1 Fig. 2.1 Development of the blastocyst; midsagittal illustrations. (A) Continued proliferation of cells produces a sphere containing a blastocystic cavity surrounded by an eccentrically located inner cell mass and a surrounding ring of trophoblast cells. (B) The inner cell mass develops further into a two-layered structure, the blastodisc, containing the epiblast adjacent to the amnionic cavity and the hypoblast adjacent to the yolk sac. (C) With further development, the blastodisc thickens cranially to form the prochordal plate. During gastrulation, cells in the epiblast migrate medially toward the primitive streak and invaginate through the primitive groove (Fig. 2.2A). The initial wave of cells displaces the hypoblast cells laterally and forms the definitive endoderm, whereas the next wave migrates between the epiblast and endoderm to form mesoderm. Cells remaining in the epiblast will spread out to replace those that have migrated through the primitive streak and will form the ectoderm (neuroectoderm and cutaneous ectoderm). During primitive streak regression, prospective notochordal cells located in the Hensen node at the cranial end of the primitive streak (Fig. 2.2B) invaginate through the primitive pit to form the midline notochordal process between the epiblast and hypoblast1,2; as gastrulation proceeds, the notochord is eventually sandwiched between the endoderm ventrally and the neuroectoderm dorsally. The notochord, together with the paraxial mesoderm, will form the vertebral column. Fig. 2.2 Normal human gastrulation. (A) Prospective endodermal and mesodermal cells of the epiblast migrate toward the primitive streak and ingress (arrows) through the primitive groove to become the definitive endoderm and mesoderm. (B) Prospective notochordal cells in the cranial margin of the Hensen node will ingress through the primitive pit during primitive streak regression to become the notochordal process. In primate embryos,3,4 the developing notochord undergoes a peculiar series of morphogenetic events between POD 17 and 25. The notochordal process initially consists of a median cord of cells radially arranged about a central lumen; the central lumen (or notochordal canal) is continuous dorsally with the amnionic cavity through the primitive pit (Fig. 2.3A). The notochordal process continues to elongate between POD 17 and 19 and reaches its full length by POD 19 to 21. It is initially rod shaped and lies between the neuroectoderm and the endoderm; however, between POD 18 and 20 it fuses, or intercalates, with the underlying endoderm to form the notochordal plate (Fig. 2.3B), bringing the notochordal canal into communication with the yolk sac. The most caudal portion of the notochordal canal is the primitive neurenteric canal, which is continuous both with the amnion through the primitive pit and with the yolk sac as a result of intercalation; this communication is called the neurenteric canal.4 The neurenteric canal remains open until POD 21 to 23 at which time the notochordal plate folds dorsoventrally and separates (or excalates) from the endoderm; by POD 23 to 25 the notochordal process has completely separated once again from the underlying endoderm (Fig. 2.3C). Thereafter, the true notochord exists as a solid rod of notochordal cells.4 Fig. 2.3 Formation of the notochord. (A) The notochordal process contains a central lumen (the notochordal canal), which is continuous with the amnionic cavity through the primitive pit. (B) During intercalation, the canalized notochordal process fuses with the underlying endoderm; the communication of the amnion with the yolk sac forms the primitive neurenteric canal. (C) During excala-tion, the notochord rolls up and separates from the endoderm to become the definitive notochord; the primitive neurenteric canal becomes obliterated. Primary neurulation involves the shaping of the neuroectoderm to form the neural plate, and bending of the neural plate to form the neural tube (Fig. 2.4). The neural plate is evident by POD 17 to 19, with the neural groove forming a midline crease immediately above the notochord. By POD 19 to 21, the neural groove has deepened considerably and neural folds are developing laterally.1 The neural folds converge toward the midline and meet to form a closed neural tube between POD 21 and 23. Closure of the neural tube is accompanied by separation of neural from cutaneous ectoderm (called dysjunction). Neural crest cells arise from the neural tube at the junction between the neural folds and adjacent surface ectoderm, and differentiate into several different cell types, including the pharyngeal arches, the meninx (pia and arachnoid), the cranial and spinal Schwann cells and melanocytes, the cranial ganglia, and the dorsal root ganglia and roots. Neural tube closure takes place over 4 to 6 days and may occur simultaneously at several initiation sites along the craniocaudal neural axis.5–8 The last portions to close are the anterior neuropore at the level of the commissural plate (closing during POD 23 to 25), and the caudal neuropore at the level of the second sacral spinal cord segment (closing during POD 25 to 27).9,10 All neural tissue cranial to the second sacral segment is therefore derived from primary neurulation. Fig. 2.4 Primary neurulation. Scanning electron micrographs showing primary neurulation in chick embryos. (A) The neural plate exists initially as a layer of pseudostratified columnar epithelium in the midline of the rostral half of the embryo. (B) The neural groove develops in the neuroectoderm overlying the underlying midline notochord, and the neural folds begin to elevate. The cutaneous ectoderm is attached laterally to the neuroectoderm. (C) Additional bending points (dorsolateral hingepoints) develop bilaterally, causing the neural folds to converge toward each other in the midline. (D) The neural folds have fused in the midline and the cutaneous ectoderm has separated to form a layer of intact skin overlying the neural tube. (From Gilbert SF. Developmental Biology. 7th ed. Sunderland, MA: Sinauer Associates 2003. Reprinted with permission.) Fig. 2.5 Secondary neurulation. Upper illustration depicts secondary neurulation in avian embryos. (A) The medullary cord consists of multiple luminae, each surrounded by an outer layer of tightly packed, radially oriented cells and containing an inner group of more loosely packed cells. (B) Adjacent cords coalesce to form larger aggregates; simultaneously, the inner cells are lost. Eventually a single structure is formed, having a single lumen that is not yet in direct communication with the lumen formed by primary neurulation. (C) Later, the neural tube (NT) formed by secondary neurulation (2° NT) fuses with that formed from primary neurulation (1° NT); at this point, the luminae of the two neural tubes communicate directly. NC, notochord. Lower illustration depicts secondary neurulation in mouse embryos. A medullary rosette is composed of cells radially arranged about an empty central lumen. The lumen is always in communication with the central canal formed by primary neurulation. Growth of the secondary neural tube occurs by additional cavitation of the secondary lumen, and by recruiting additional cells from the caudal cell mass (CCM). (From McLone DG, Dias MS. Normal and abnormal embryology of the nervous system. In: Cheek WR, ed, Pediatric Neurosurgery, Surgery of the Developing Nervous System. 3rd ed. Philadelphia: WB Saunders; 1994:3–39. Reprinted with permission.) The caudal neural tube (including the tip of the conus medullaris [CM] caudal to the second sacral segment, as well as the filum terminale) arises by secondary neurulation from the caudal cell mass or end bud (the remnants of the Hensen node and primitive streak) at the caudal pole of the embryo (Fig. 2.5). Secondary neurulation is species specific—for example, in chick embryos secondary neurulation involves the formation and subsequent coalescence of multiple independent tubules into a caudal neural tube that eventually fuses with the primary neural tube in an area called the overlap zone.11 In contrast, secondary neurulation in mouse embryos involves the progressive extension of the primary neural tube through the addition of cells onto its caudal end.12 It is unclear whether humans more closely resemble a mouse or a chicken. Ascent of the CM is a process whereby the conus changes position with respect to the surrounding vertebral column, appearing to rise or ascend over time such that spinal cord segments come to lie opposite more cranial vertebral segments, and the spinal nerves take a progressively more caudal course as they travel from their origins on the spinal cord to their respective neural foraminae (and forming the cauda equina). Ascent begins on POD 42 and continues throughout embryogenesis and perhaps even into the postnatal period. Ascent occurs through two mechanisms. The first, occurring between POD 42 and 54, is an ill-defined process referred to as retrogressive differentiation, during which the caudal neural tube becomes thinner, develops a rudimentary marginal and no mantle zone, and looks “less well developed.” Beyond POD 54, retrogressive differentiation has ceased, and all further ascent occurs because the spinal cord is elongating more slowly than the surrounding vertebral column. The rate of ascent is steeper between gestational weeks 12 and 20 and slows thereafter until term. According to Barson, the CM at birth lies opposite the L2/3 disk space and ascends to its final level opposite the L1/2 disk space by 2 months postnatal.13 However, several subsequent radiographic studies have demonstrated that the conus already lies opposite the L1/2 disk space at term.14,15 In a recent study of 100 children undergoing whole spine magnetic resonance imaging (MRI) scans (to evaluate for leptomeningeal seeding from tumors), the average level of the normal CM was opposite the inferior third of the L1 vertebral body, the mode was opposite the L1/2 disk space, and the lowest normal level (95% confidence limits) was opposite the middle third of the second lumbar vertebral segment.16 Therefore, any CM that is positioned caudal to the midbody of the second lumbar segment should be considered radiographically tethered. The following is a classification of central nervous system (CNS) malformations according to their reputed embryogenetic mechanism(s), keeping in mind that few of these have been validated experimentally (Table 2.1). Myelomeningocele (MMC) and anencephaly represent a simple localized failure of a portion of the neural tube to close, yielding an open or exposed malformation on the dorsum of the child. Because MMC and anencephaly reflect a failure of neural tube closure they are, by definition, open malformations still attached circumferentially to the surrounding cutaneous ectoderm (skin). The pathogenesis of MMC and anencephaly involves both genetic and environmental factors; attention has focused largely on the contributions of folate. Folate antagonists can cause neural tube defects (NTDs) both experimentally and clinically, and periconceptional folate supplementation has been shown to reduce NTDs by as much as 80%.17–19 Further studies have begun to elucidate the importance of folate within the “methylation cycle” and the conversion of homocysteine to methionine. Table 2.1 Embryologic Classification of Dysraphic Spinal Malformations
Normal Early Human Neural Development
Blastogenesis
Gastrulation and Formation of Germ Layers
Intercalation and Excalation of the Notochordal Process
Formation of the Neural Tube: Primary Neurulation
Formation of the Caudal Conus Medullaris and Filum Terminale: Secondary Neurulation
Ascent of the Conus Medullaris
Abnormal Human Early Neural Development
Failure of Neural Tube Closure: Myelomeningocele, Anencephaly
Disordered midline axial integration during gastrulation |
• Split cord malformations |
• Combined spina bifida |
• Neurenteric cysts |
• Some myelomeningoceles |
• Some cervical myelomeningoceles |
• Hemimyelomeningoceles |
• Some examples of caudal agenesis and Klippel-Feil |
• Complex dysraphic malformations |
Localized failure of neurulation |
• Myelomeningocele |
• Anencephaly |
Premature ectodermal dysjunction |
• Lipomyelomeningoceles |
Incomplete ectodermal dysjunction |
• Dermal sinus tracts, dermoid/epidermoid tumors |
• Meningocele manqué? |
• Meningocele? |
Disordered formation of the caudal cell mass or secondary neurulation |
• Terminal spinal lipomas |
• Fatty filum terminale |
• Myelocystoceles |
Postneurulation disorders (not discussed) |
• Encephaloceles |
• Chiari II malformation |
The genesis of cervical and upper thoracic MMC likely involves a different embryonic mechanism.20,21 Whereas lumbosacral MMCs are open, have a larger fascial defect, and are commonly associated with a complete loss of sensorimotor function below the level of the malformation, cervicothoracic lesions are usually closed, have a limited fascial defect, and are associated with little or no loss of sensorimotor function below the malformation. Approximately 50% of cervicothoracic malformations are associated with split cord malformations (see below). These more cranial lesions have been hypothesized to arise through a limited failure of the final stages of neural tube closure, through myelocystocele formation21,22 (see below), or through an abnormality of gastrulation.23
Anomalies Resulting from Incomplete Dysjunction: Dermal Sinus Tracts, Meningoceles Manqué, and Meningoceles
Several malformations—dermal sinus tracts, meningoceles manqué, and perhaps even true meningoceles—may represent disorders of dysjunction in which a persistent tract of tissue, variably containing epidermal or dermal elements, fibrous tissue, and/or peripheral nerve elements (nerve roots and/or ganglion cells) extends a variable length from the dorsum of the spinal cord toward the skin.24 Dermal sinus tracts (DSTs) incorporate a tract of cutaneous ectoderm (with or without fibrous tissue) that extends from the dorsal midline skin to the neural tube. Approximately 60% of dermal sinuses incorporate a dermoid or epidermoid tumor; conversely, 30% of dermoid and epidermoid tumors occur in association with DSTs.25,26 The proposed embryogenesis involves faulty separation of neuroectoderm from cutaneous ectoderm at the time of dysjunction,27 most frequently involving the posterior neuropore (Fig. 2.6). Accordingly (and importantly from a surgical perspective), the intradural tract usually ends on the dorsum of the CM at the level of the second sacral segment, cranial to the tip of the CM and separate from the origin of the filum terminale.
Meningocele manqué (“failed” meningocele) was a term originally used by James and Lassman to describe a malformation characterized by a small scarified lesion (sometimes referred to as a “cigarette burn”) on the skin, having an underlying fibrous tract (and, in their original description, aberrant nerve roots) that extends a variable distance from the spinal cord to the skin.28 Although originally described as atretic or abortive meningoceles, these malformations likely represent another example of disordered dysjunction in which the cutaneous ectoderm, rather than being displaced toward the spinal cord, instead incorporates fibrous tissue and/or neural crest cells that are drawn up toward the surface ectoderm. Approximately half have been described in association with split cord malformations28 and may represent a secondary effect of disordered gastrulation.23
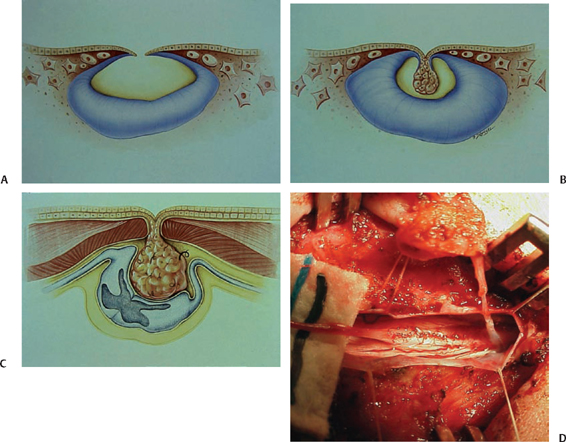
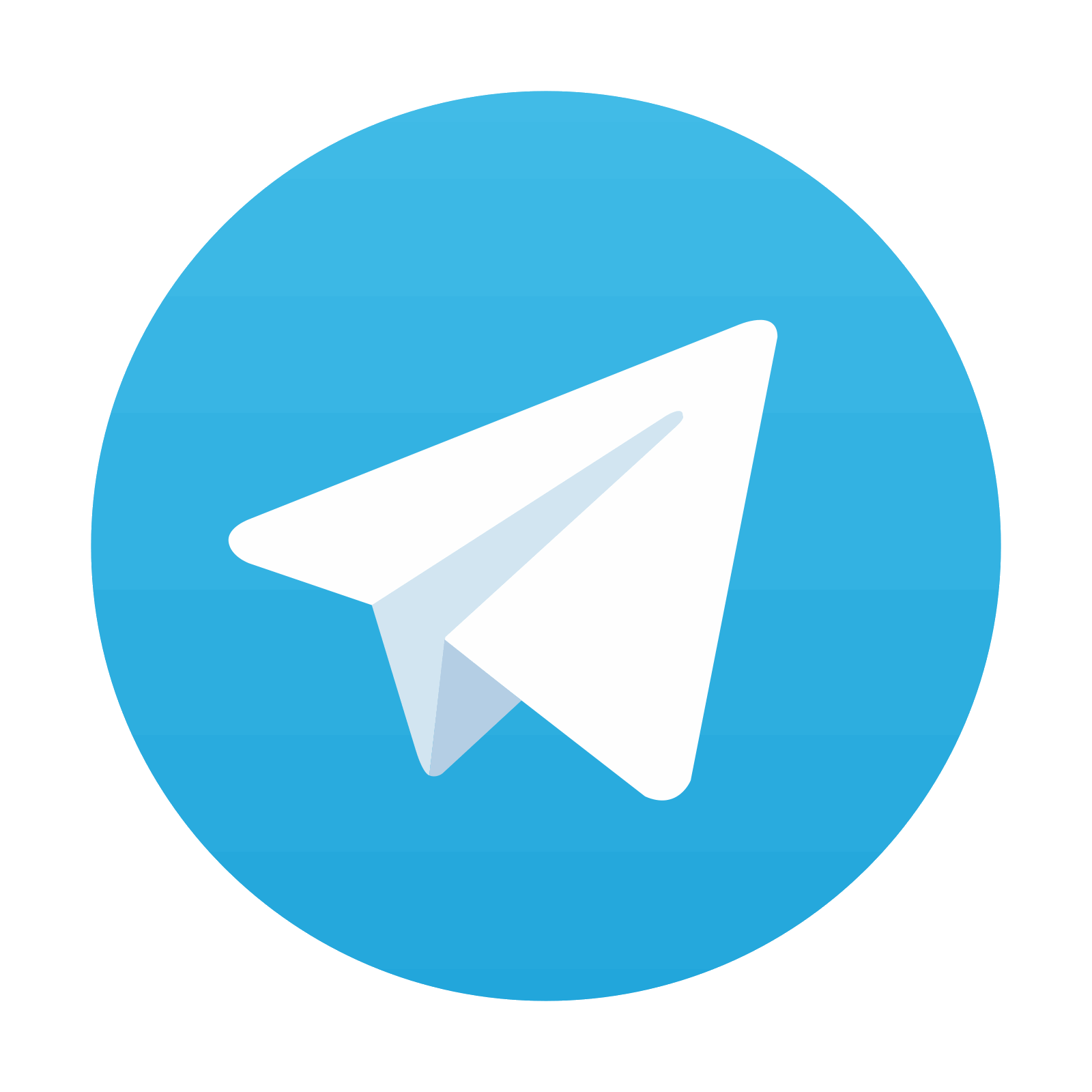
Stay updated, free articles. Join our Telegram channel
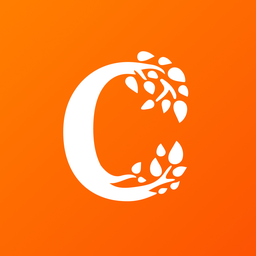
Full access? Get Clinical Tree
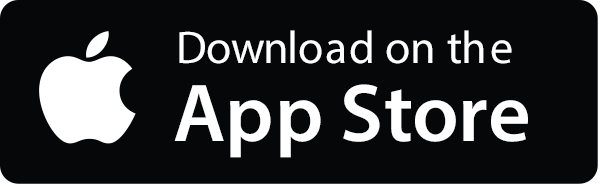
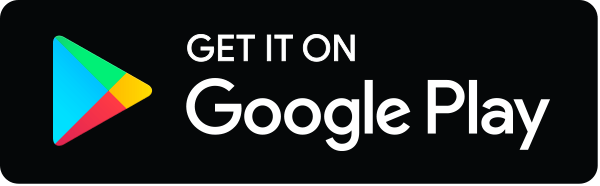