Neurotransmitter/neuromodulator
Location of the soma
Neuronal pattern of discharge
Wakefulness promoting
Orexin
Postero-lateral hypothalamus
Wake-on
Acetylcholine
LDT-PPT and BFB
Wake/REM-on
Serotonin
DRN and MRN
Wake-on
Noradrenaline
Locus coeruleus
Wake-on
Dopamine
VTA and SNPc
Bursting discharge during wakefulness and REM sleepb
Histamine
TMN
Wake-on
GABAa
NPO
Probably Wake-on
Glutamatea
Mesopontine reticular formation
Probably Wake-on
NREM sleep promoting
Melanin-concentrating hormone (MCH)
Postero-lateral hypothalamus
Active in NREM and tonic REM
Adenosine
Probably BFB, POA
c
GABAa
VLPO, MnPO
NREM-on and NREM-REM-on
REM sleep promoting
Acetylcholine
LDT-PPT and BFB
Wake/REM-on and REM-on
Melanin-concentrating hormone (MCH)
Postero-lateral hypothalamus
Active in tonic REM sleep
Orexin
Postero-lateral hypothalamus
Active in phasic REM sleep
GABAa
vlPAG, DRN
Probably REM-on
Glutamatea
NPO
Probably Wake/REM-on
3 Orexinergic Neurons, Orexins and Receptors
Orexin-A and B (also called hypocretin-1 and 2) were discovered in 1998 by two independent groups (de Lecea et al. 1998; Sakurai et al. 1998). These neuropeptides are synthesized by a discrete group of neurons (~5000 in rodents, ~11,000 in cats and 20–50,000 in humans) in the postero-lateral hypothalamus (Li et al. 2013; Torterolo et al. 2006). Figure 1 shows the characteristics and distribution of the orexinergic neurons in the postero-lateral hypothalamus of the guinea pig. Orexins exert their biological function through two metabotropic receptors orexin-R1 and orexin-R2 (also known as hypocretin 1 and 2 receptors) that have broad, partially overlapping, but distinct patterns of distribution throughout the brain and body. The orexin-R1 has 10 to 100 times more affinity for orexin-A than for orexin-B; in contrast, orexin-R2 has the same affinity for both neuropeptides. Through these receptors, orexins produce an excitatory effect at postsynaptic sites (Mignot 2011; Li et al. 2013; van den Pol and Acuna-Goycolea 2006). It has been demonstrated that orexin increases Na+-dependent current, non selective cation currents, and activates the Na+/Ca++ exchanger. Depression of K+ currents and increase in intracellular Ca++ was also demonstrated. At presynaptic sites orexin also increases the neurotransmitter release.
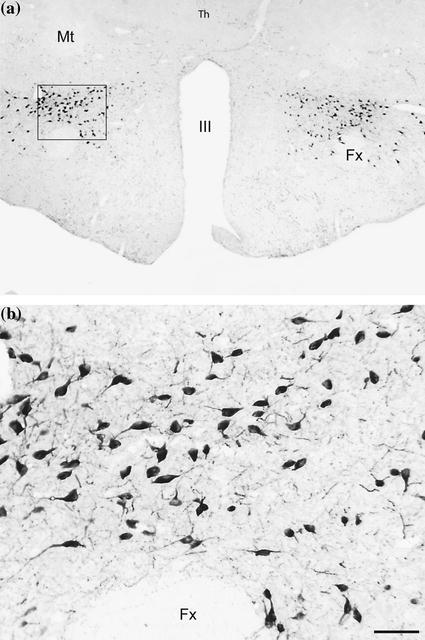
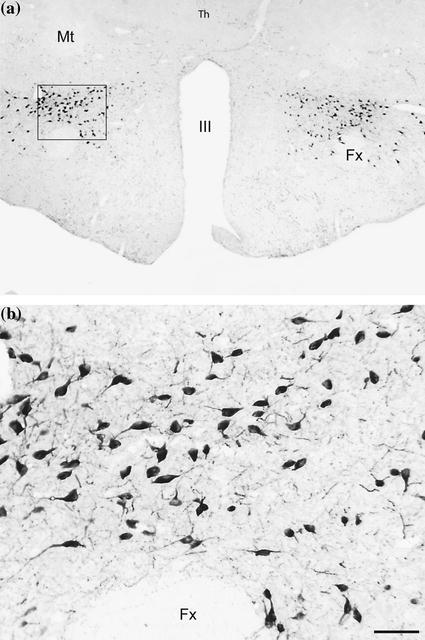
Fig. 1
Orexinergic neurons are located in the postero-lateral hypothalamus. a Photomicrograph that shows the distribution of the orexinergic neurons in a coronal plane of the tuberal region of a guinea pig hypothalamus. b The inset showed in (a), is exhibited in a higher magnification in (b). The orexinergic neurons are located close to the fornix. Calibration bar, 50 μm. Sections of 20 μm were processed employing the anti-orexin-B antibodies, ABC method and the DAB-H2O2 reaction to detect peroxidase activity
4 Projections of the Orexinergic Neurons
Orexinergic neurons project throughout the central nervous system (CNS) (Peyron et al. 1998); furthermore, the activity of the peripheral organs is also influenced by the orexins (Voisin et al. 2003). Orexinergic neurons have also the potential to mediate complex functions since they exhibit the morphology of prototypical “command” neurons, which are small groups of highly specialized cells that coordinate and integrate, in a complementary fashion, the activities of a vast number of neural and hormonal systems (Torterolo and Chase 2014).
Sensory and motor nuclei are directly innervated by orexinergic neurons (McGregor et al. 2005; Torterolo et al. 2007; Fung et al. 2001; Yamuy et al. 2004). Orexinergic neurons also project to the thalamus and cortex (Peyron et al. 1998), wherein they directly influence thalamo-cortical activities that support cognitive functions. Furthermore, dense concentrations of orexin-containing axon terminals are located in the TMN (Eriksson et al. 2001) as well as in brainstem areas such as the LDT-PPT, VTA, LC and DRN (Peyron et al. 1998; Chemelli et al. 1999; Date et al. 1999; Nambu et al. 1999), that participate in the control of wakefulness and REM sleep. We have demonstrated also that orexinergic neurons project to the NPO, that exerts executive control over the initiation and maintenance of REM sleep (Torterolo et al. 2013). Figure 2 shows orexinergic projections toward the LDT-PPT, LC and DRN in the cat.
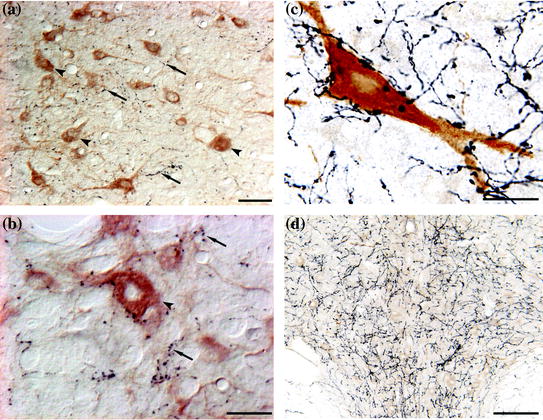
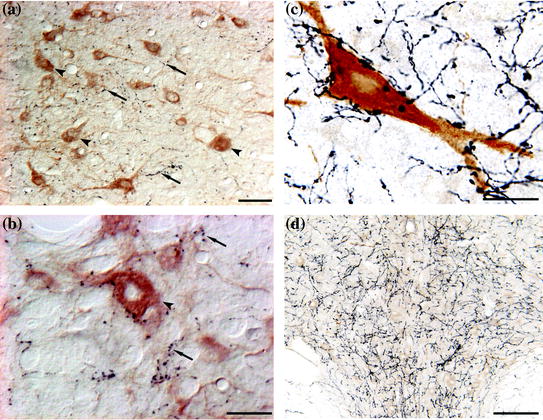
Fig. 2
Examples of the orexinergic projections. a and b Photomicrographs of the LDT-PPT of the cat. The sections were immunostained for orexin (in black, examples of orexienergic fibers/terminals are indicated by arrows) and for choline acetyltransferase (in brown, examples of cholinergic neurons are signaled by arrowheads). Sections were processed utilizing the ABC method and the DAB-H2O2 reaction to detect peroxidase activity. This reaction was enhanced with nickel to label orexinergic cells. Calibration bars a and b, 30 and 20 μm respectively. c Photomicrographs of the LC of the cat. The sections were immunostained for orexin (in black) and tyrosine hydroxylase (in brown). Sections were processed utilizing the ABC method and the DAB-H2O2 reaction to detect peroxidase activity. This reaction was enhanced with nickel to label orexinergic cells. Calibration bar 10 μm. d The photomicrograph shows the orexinergic fibers in the dorsal raphe nucleus; this is taken for another section of the experimental series shown in (c). Calibration bar 100 μm (Color figure online)
Orexinergic neurons also project to the NREM generation areas such as the preoptic area (Peyron et al. 1998).
5 Orexinergic Neurons as a Part of the Activating Systems
The postero-lateral hypothalamic area where the orexinergic neurons are located, is the key brain site that, for decades, has been identified as being responsible for initiating, coordinating and maintaining goal-oriented survival-type behaviors such as fight, flight and food consumption among others. In addition, and in accord with the preceding concept, experimental studies have demonstrated that this area is critically involved in the control of sleep and wakefulness, somatomotor activity as well as “pleasure” or “reward” (Torterolo and Chase 2014; Chase 2013).
Early studies revealed that the intraventricular injection of orexin induces wakefulness (Hagan et al. 1999; Piper et al. 2000). These data, as well as the fact that the lack of orexinergic neurons in narcoleptic patients induces hypersomnia (Mignot 2011), strongly suggested that this system promotes wakefulness. Genetically modified mice and optogenetic studies also confirmed the role of orexin and orexinergic neurons as a waking promoting substance and system (Chemelli et al. 1999; Hara et al. 2001; Adamantidis et al. 2007). However, by means of Fos technology (the Fos protein is a marker of neuronal activity), we demonstrated in the cat that orexinergic neurons are not active during wakefulness per se (Torterolo et al. 2001) (Fig. 3). A detailed analysis of orexinergic neuronal activity shows that these neurons are strongly activated when animals are exploring an unknown environment (exploratory motor activity) (Torterolo et al. 2011). In the absence of motor activity during alert wakefulness, quiet wakefulness or quiet sleep, the orexinergic neurons are not activated to any significant extent (Torterolo et al. 2003). In fact, the number of Orexin+ Fos+ neurons was approximately 10 times greater during exploratory motor activity than during repetitive motor activity that occurred during forced locomotion, even though in both paradigms there was a comparable amount of motor activity (Torterolo et al. 2011). Therefore, neither wakefulness nor motor activity per se, were critical with respect to the activation of orexinergic neurons. Hence the orexinergic system is engaged when animals are performing goal (reward)-directed behaviors. In agreement with our results, it was found that orexin knock-out mice were unable to work for food or water reward during the light phase (McGregor et al. 2011).
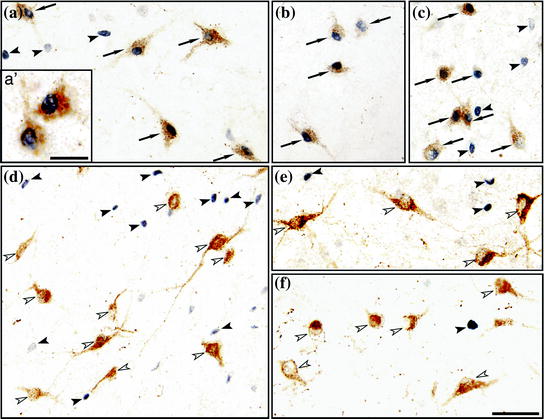
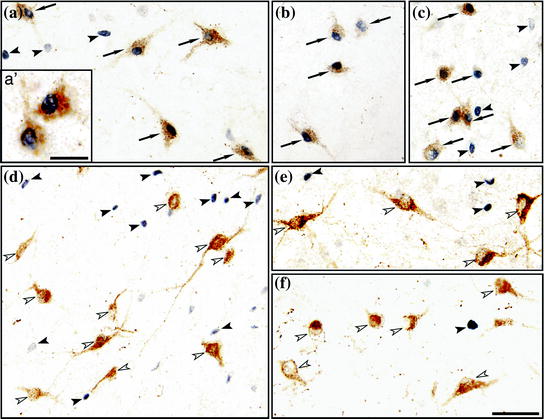
Fig. 3
Photomicrographs containing orexin and Fos immunoreactive neurons in the lateral hypothalamus of the cat during active wakefulness with motor explorative activity (a, a′, b and c), alert wakefulness without motor activity (d), quiet wakefulness (e) and NREM sleep (f). Orexinergic neurons are stained brown; Fos immunoreactivity, that is restricted to nuclei, is shown in black. Arrows indicate Orexin+ Fos+ cells; these neurons are exhibited with higher magnification in a′. Significant numbers of Orexin+ Fos+ neurons were observed only during active wakefulness with motor explorative activity. Filled arrowheads point to non-orexinergic Fos+ neurons, these neurons were observed in all of these behavioral states. Empty arrowheads indicate orexinergic neurons that did not express c-fos. Calibration bars a–f 50 μm; a′ 20 μm. Modified from Torterolo et al. (2003) (Color figure online)
Recently, Chase presented the “Unified Survival Theory for the Functioning of the Orexinergic System” (Chase 2013). The basis of this theory is that the main role of the orexinergic system is to initiate, coordinate and maintain survival behaviors and survival-related processes.
In order to promote these waking effects during survival-related behaviors, orexinergic neurons activate different components of the activating systems such as the LC, DRN, LDT-PPT, TMN and BFB.
6 Orexinergic Neurons During Sleep
6.1 NREM Sleep
The orexinergic neurons, as a component of the activating systems (Torterolo and Vanini 2010a), do not actively participate in the occurrence of NREM sleep. In fact, there is a lack of Fos expression in these neurons during this behavioral state (Fig. 3). Subsequently, unit recordings confirmed our early findings (Mileykovskiy et al. 2005; Lee et al. 2005; Takahashi et al. 2008; Kolaj et al. 2008).
6.2 “Tonic” REM Sleep
The orexinergic neurons are considered to be REM-off neurons. The REM-off profile concept of the orexinergic neurons arose based upon electrophysiological recordings of identified orexinergic neurons during “tonic” REM sleep (see below) (Mileykovskiy et al. 2005; Lee et al. 2005; Takahashi et al. 2008).
6.3 “Phasic” REM Sleep
EEG activation, theta activity in the hippocampus and muscle atonia are the classic biomarkers for the identification of REM sleep. Accompanying these “tonic” signs are rapid-eye movements, muscle twitches, PGO waves, breathing irregularities as well as heart rate and blood pressure variations that constitute the phasic events of REM sleep. Other signs such as acceleration of the theta rhythm also correlate with these phasic events (Rowe et al. 1999).
Experimental evidence shows that while orexinergic neurons turn off during “tonic” REM sleep, at least a subset of these neurons discharge in bursts during phasic REM sleep (Mileykovskiy et al. 2005; Lee et al. 2005; Takahashi et al. 2008).
Studies in the cat, an animal that exhibits robust phasic periods of REM sleep (Ursin and Sterman 1981), strongly suggest that there is orexinergic neuronal activity during REM sleep, probably during the phasic events of this state. An increase in the number of Orexin+ Fos+ neurons was observed during REM sleep induced by carbachol microinjections into NPO (Torterolo et al. 2001). In this study, REM sleep was induced by carbachol microinjections in order to generate a state of sufficiently long duration to allow Fos protein to be synthesized in high concentration. During this state, 34 % of the orexinergic neurons were activated according to their Fos-expression (Torterolo et al. 2001). This result was in agreement with the findings by Kiyashchenko et al. (2002), who described an increase in orexin-A release during REM sleep, both in the hypothalamus and BFB in freely moving cats. Hence, this study also indicates that orexinergic neurons are active during REM sleep, probably in conjunction with phasic events.
Microinjection studies suggest also that administration of orexin into critical areas such as the medullary reticular formation of the rat or the NPO of the cat, can induce REM sleep-like atonia or REM sleep, respectively (Mileykovskiy et al. 2002; Xi et al. 2002; Xi and Chase 2010).
If orexinergic neurons are active during “phasic” REM sleep, they are likely to promote the phasic events of REM sleep (Torterolo and Chase 2014). In fact, orexins directly activate motor nuclei, breathing neuronal networks and sympathetic output that controls the cardiovascular system (Yamuy et al. 2004; Shirasaka et al. 2002; Zhang et al. 2005; Williams and Burdakov 2008), as well as the medial septum where the pacemaker for the hypocamppal theta rhythm is located (Gerashchenko et al. 2001). These are typical ergotropic or energy-expending behaviors that are induced by the orexinergic system (Chase 2013).
Then, orexinergic cells in the hypothalamus may be involved in the control of both active wakefulness and active components of REM sleep. As mentioned above, the orexinergic system plays an important role in the regulation of motor activity during motivational states. Is it possible that this system promotes motor activity during wakefulness and atonia during REM sleep? Actually, this pattern of duality of behavioral state control with opposite motor responses is reminiscent of the phenomenon of reticular response-reversal in the NPO (Chase and Babb 1973; Chase and Morales 1990). This phenomenon involves mechanisms that result in the facilitation of wakefulness and somatomotor activation during wakefulness, as well as the generation of REM sleep and its accompanying pattern of motor inhibition during this sleep state. The reticular response reversal determines the auditory stimulation promotion of somatomotor activation during wakefulness, while increasing the hyperpolarization of the motoneurons and atonia during REM sleep (Chase 2013). In fact, microinjections of orexin-A or orexin-B into the NPO of the cat increases the time spent in REM sleep and results in a decrease in the latency to the generation of this state (Xi et al. 2002). Juxtacellular application of orexin-A results in an increase in the excitability of NPO neurons, which is associated with the induction of REM sleep (Xi et al. 2003). In addition, orexin-A increases acetylcholine release in the NPO of the rat (Bernard et al. 2003, 2006). In this respect, it is known that acetylcholine levels within this region increase during REM sleep (Kodama et al. 1990). On the other hand, an increase in wakefulness accompanied by a decrease in REM sleep has been observed also when orexin-A is microinjected into the NPO of the cat (Moreno-Balandran et al. 2008). Furthermore, the iontophoretic application of orexin-A into the NPO of the rat produces an inhibition of NPO neurons, which can be blocked by previous iontophoretic application of bicuculline, a GABAA receptor antagonist (Nunez et al. 2006). In fact, it has been shown that orexin increases GABA levels in the NPO of the rat, and orexin and GABA interact within this nucleus to promote wakefulness (Watson et al. 2008; Brevig et al. 2011). The presence of orexin-B receptors on GABAergic neurons within the NPO may be the cellular basis for this effect (Brischoux et al. 2008). The paradoxical or contradictory findings involving the REM sleep and wakefulness promoting actions of orexin within the NPO have been reconciled by Xi and Chase (Xi and Chase 2010). They demonstrated that the microinjections of orexin-A within the NPO generates REM sleep when applied during NREM sleep, but promotes wakefulness when applied during this behavioral state. Thus, the behavioral state of the animal at the time of the application of orexin determines whether REM sleep or wakefulness occur.
7 Afferents to Orexinergic Neurons
Orexinergic neurons receive inputs from several regions such as the allocortex, many hypothalamic nuclei, the periaqueductal gray matter, the DRN, and parabrachial regions, which suggests that these neurons integrate a variety of interoceptive and homeostatic signals (Yoshida et al. 2006). Interestingly, these neurons seem to be weakly influenced by exteroceptive sensory inputs (Mileykovskiy et al. 2005).
8 Effects of Different Neurotransmitters on Orexinergic Neurons
Table 2 summarizes the effects of the main neurotransmitters on orexinergic neurons. In mice, glutamate depolarizes orexinergic neurons acting through AMPA and NMDA receptors, while GABA inhibits these neurons though GABAA and GABAB receptors (Yamanaka 2006).
Table 2
Examples of the effects of neurotransmitter/neuromodulators onto orexinergic neurons (in vitro studies)
Neurotransmitter/neuromodulator | Cellular effects on orexineric neuron | References | Animal model |
---|---|---|---|
Orexin | despolarization by presynatic facilitation of glutamate release | Li et al. (2002) | Mouse |
Acetylcholine | Carbachol depolarizes 27 % and hyperpolarizes 6 % of the neurons | Yamanaka (2006) | Mouse |
Acetylcholine has a predominantly excitatory effect | Bayer et al. (2005) | Rat | |
Serotonin | Hyperpolarizes through 5HT1A receptors | Yamanaka (2006) | Mouse |
Noradrenaline | – Hyperpolarizes through α2 receptors | Yamanaka (2006) | Mouse |
– Depolarization mediated by α1 receptor (in the presence of a α2-receptor antagonist) | Yamanaka (2006) | Mouse | |
– Predominant excitatory effect | Bayer et al. (2005) | Rat | |
Dopamine | – D1 and D2 dopamine receptors have opposing effects on excitatory presynaptic terminals that impinge on orexinergic neurons | Alberto et al. (2006) | Rat |
Histamine | – Almost no effect | Yamanaka (2006) | Mouse |
GABA | Post-synaptic inhibition though GABAA and GABAB receptors | Yamanaka (2006) | Rat |
Glutamate | Depolarize acting through AMPA and NMDA receptors | Yamanaka (2006) | Mouse |
Melanin-concentrating hormone (MCH) | Attenuates orexin-A induced enhancement of spike frequency and the frequency of miniature excitatory postsynaptic currents | Rao et al. (2008) | Mouse |
Adenosine | depresses the amplitude of evoked excitatory postsynaptic potential and the frequency of spontaneous and miniature excitatory postsynaptic currents | Liu and Gao (2007) | Mouse
![]() Stay updated, free articles. Join our Telegram channel![]() Full access? Get Clinical Tree![]() ![]() ![]() |