Fig. 1
a Commonly used pulse forms. The depolarization pulse is for all pulse forms rectangular with a pulse-width up to 300
s and the hyperpolarization pulse is (a) not existent = monophasic pulse; b the same as depolarization pulse = symmetric biphasic pulse; c longer than the depolarization pulse, with a subthreshold amplitude = asymmetric rectangular biphasic pulse; or d shorter than the depolarization pulse, with a subthreshold pulse duration = asymmetric exponentially decreasing biphasic pulse

Stimulation frequency
A stimulation pulse produces in the muscle a twitch that fades out after 200 ms (see Fig. 2). When the stimulation signal is a train of pulses, the ratio between this muscle relaxation time and the period between pulses is crucial to understand the response of the muscle, which is highly dependent on the stimulation frequency.
When the stimulation frequency is below 10 pulses per second (10 Hz), the muscle twitches can still be differentiated from each other, and the muscle response can be characterized as a sort of tremor. This effect gradually decreases when the stimulation frequency increases, usually around the band of 10–30 Hz, until it reaches a point where it evokes a smoother muscle response known as tetanic contraction (see Fig. 2). The frequency when this happens is called the fusion frequency, and its exact value varies between subjects and fibers.
However, this does not mean that functional electrical stimulation should always use high frequencies. In fact, when the stimulation frequency keeps increasing toward the fusion frequency and above, the muscles start to fatigue very fast. The main reason is that with FES the excited fibers are all firing synchronously and not asynchronously and distributed among several hundreds to thousand fibers as it is the case in the natural activation. In the natural case each single fiber is only activated 0.3–5 times per second allowing sufficient recovery time for recovery and energy uptake. In FES the stimulation frequencies that are normally used are in the range from 20 to 100 Hz, depending on the application.
Stimulation amplitude
Changes in the stimulation amplitude or pulse duration cause similar effects on the elicited muscle response. Higher stimulus intensities produce higher contractions through activation of more fibers, which can be measured as higher joint torques. However, there is a lower threshold under which the stimulus produces no response, and also an upper threshold of saturation where no additional fibers can be recruited. Additionally, the muscle response between the two thresholds is not linear; as it has been described before, the electrically-induced response of the muscle is created by the activation of several muscle fibers whose activation threshold depends on their diameter. Smaller myelinated nerves have closer nodes of Ranvier, which means that to elicit an action potential using FES we would need a higher voltage gradient (thus, a higher electric field and a higher current). Finally, the distance between the muscle fibers and the skin also plays a fundamental role, since the fibers closer to the electrode are reached by a stronger electric field.
It could be shown in [3] that due to afferent feedback in stroke subjects higher torques in triceps muscles could only be generated with shorter pulses, as a longer pulse duration caused antagonistic muscle responses of the biceps muscles. The curves in Fig. 3 represent the pairs of intensity current and pulse-width that elicit the same motor response in a subject, and as a rule of thumb, larger pulse-widths need to be paired with lower currents in order to obtain the same motor responses. However, there is a saturation for the pulse-width that prevents the muscle from producing a stronger contraction, even when the current amplitude is maintained and the pulse width is increased. The stimulated motor response in healthy subjects is in between the innervated motor threshold and the maximum motor response; therefore, the dotted rectangle represents the range that is normally used in FES applications. For example, for wrist extension the typical pulse widths vary from 40 to 300
s, whereas the current amplitude is usually set up between 10 and 40 mA. However, it is very dependent on the subject pain threshold and the muscle that has to be activated. The other extreme is completely denervated muscles, e.g., in spinal cord injured patients. Those muscles are no longer connected to the spinal cord (e.g., a cauda equina lesion) and get completely denervated. In such cases, it is possible to produce, after a long training period of weeks to months, muscle contractions by direct stimulation of the motor end plate instead of the nerve. However, the pulse-widths that must be used have to be 100 times larger. Here, one cannot increase the amplitude instead, as the high currents would heat the tissue to unaccepted levels.
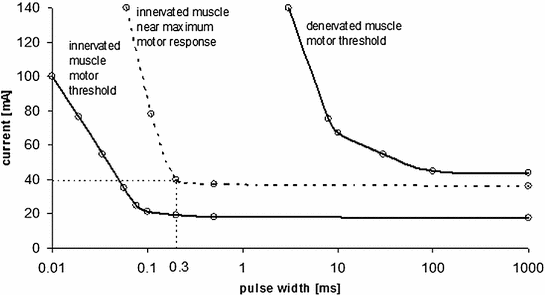

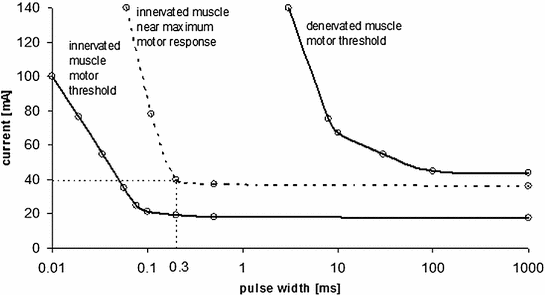
Fig. 3
Curves of equal motor response for different pulse widths and pulse amplitudes. For intact motor neurons stimulation pulses longer than 300
s do not increase the motor response if the stimulation amplitudes are higher than 40 mA. Denervated muscles require a 100–1000 times longer stimulation pulse-widths than innervated muscles (Data from wrist extensor, surface stimulation, stimulation frequency 35 Hz). Adapted from [3]

Electrode placement
Two main aspects must be considered for the electrodes placement: the position and the distance between anode and cathode.
In order to elicit muscle responses, the active electrode (cathode) must be placed on the muscle belly, close to the innervating nerve or anywhere else where the innervating nerve is close to the surface. Placing them on the muscle belly is easier, especially in the case of the lower limbs, where the muscles are bigger and the electrodes must simply be placed along the fiber. Alternatively, the stimulation of the innervating nerve at some other location (where this nerve is close to surface) has the advantages that stimulating deeper lying muscles can be reached. Often smaller electrodes are used in this case, which sometimes can have the drawback of making the subject feel discomfort. The area that can be found to stimulate a specific muscle is called the motor point of this muscle. Some regions of the body have several motor points very close, like the arm or the hand. For such regions, the best option is to use multiple small electrodes as proposed with electrode arrays.
The second aspect that plays an important role is the distance between the anode and cathode. Considering the tissue to be homogeneous, the same electrical potential difference between the electrodes will create a stronger and more superficial electrical field if the electrodes are closer. This electrical field will activate only the superficial fibers, and with a very high intensity, which can be useful for small muscles (like the arm muscles). On the other hand, bigger muscles (like for instance the rectus femoris) require a higher distance between electrodes, where the electrical field will reach more fibers with a lower intensity. However, there is a drawback for increasing the distance between electrodes: as the electrical field spreads out over the tissue, it can activate other muscles, including the antagonists if the distance is high enough.
Electrode size
The choice of the size of the electrode (i.e., the size of their active area) depends on the dimension of the muscle or nerve that wants to be activated. As a rule of thumb, larger electrodes will reach deeper nerves, thus achieve overall stronger contractions but have a lower muscle selectivity. Small electrodes can result in very high current densities, which can lead to skin irritations or burns. Generally, small electrodes provide a better selectivity, however limit the penetration depth. The size of the electrodes that are normally used ranges between 2 and 50 cm
; the smallest ones are used for stimulating superficial nerves, those of 6–10 cm
are indicated for smaller muscles, whereas electrodes bigger than 25 cm
or more are designed for larger muscles or dermatomes [1].



2.3 Modeling FES
The recent development of more selective FES technologies has created the need to better understand the properties of the nerve, bone, muscle, fat, and skin, and how they react when external electrical stimuli are applied. In this scope, simulations of body segment volume conductor models have been used to determine which structures are important to be considered and how stimulation parameters need to be optimized for more efficient stimulation of neural structures. This second aspect requires a two-step model, the volume conductor with all relevant physical properties and a nerve model that determines when the neuronal structure is activated. Simpler, more classical modeling of effects of electrical stimulation to tissues can be done with electrical circuit models, in case the physical structures and anatomical properties are not important.
2.3.1 Models Used for Describing the Electrical Effects of Electrical Stimulation
Both modeling approaches characterize the full dynamics that connects the stimulation current to the nerve recruitment. In this section we first describe simplified one-dimensional electrical models and then the combination of volume conductor models with nerve activation models.
Simplified electrical models
The simplest way of modeling the skin and underlying tissues is by assuming their permittivity and resistivity is homogeneous and hypothesizing the propagation of the current impulse to occur only along one axis. This leads to the equivalent electrical model that is shown in Fig. 4, consisting on a resistor representing the fat and the muscle (Rc) in series with a RC parallel circuit representing the skin (Cs, Rs), and an additional capacitor and resistance that model the electrode polarization effects, which can be neglected for surface FES. The values of the body-core resistance, and the skin resistance and capacitance can be experimentally determined by current–voltage responses measured on the skin and with needle electrodes within the muscle tissue. Results show that the value of the body-core resistance is independent of the current density, whereas the skin resistance decreases when the stimulation amplitude increases using a specific stimulation electrode size (see Fig. 4). Hence, the skin impedance is a non-linear function of the current density [6].
Volume conductor models
A full characterization of the three-dimensional propagation of the stimulation current and the induced voltage gradients requires a more advanced model that includes more complex volumetric models, where current and voltage can have arbitrary directions. Recent studies have used a finite element (FE) model to simulate the distribution of the potential inside the biological tissues, i.e., the skin, fat, muscle and bone layers [9]. These types of models can work with detailed geometries (reconstructed from MRI scans), are able to deal with inhomogeneities (such as sweat ducts and glands), and can simulate specific stimulation pulse waveforms, electrode positions and sizes. The equations that are used to model the propagation of the electrical field can be static or quasi-static or transient (including the inductive effects and the times of wave propagation). Additionally, each tissue layer can be defined in terms of geometry and structure, and its electrical properties can also be accurately specified (permittivity, conductivity). The inclusion of the dielectric properties of the tissues was introduced by Kuhn and colleagues [9], proving that the differences in the dielectric properties between tissue layers create capacitive effects that are not negligible for the skin. Their method obtained very good comparisons with experimental measurements on healthy volunteers.
Nerve models
The second step of modeling tissue excitability focuses on the transmembrane potentials of the nerve axons, which depend on the extracellular potentials that are calculated using the FE model of the conductor volume. The nerve model contains thousands of myelinated axons that can be activated using electrical current pulses, generating action potentials that activate the muscle fibers [12]. The main information that can be extracted from the nerve models is which factors are important in the design of the optimal transcutaneous electrical stimulation.
2.3.2 Important Factors for Nerve Activation
The analysis and simulations with these two-step models can be used to extract quantitative relations between the several factors and the stimulation outcome. The main factors that have been investigated with respect to the volume geometry were thicknesses, nerve depth, inhomogeneities, electrode position, electrode size, properties of the different layers (permittivity, resistivity), modeling equations (transient or quasi-static, including the pulse amplitude and duration), and the type of nerve model (axon diameter and diameter distribution) [9, 11, 12]. The parameters that have a stronger influence in transcutaneous electrical stimulation are the nerve depth, the resistive properties of the muscles, the axon diameters, and the axon diameter distribution. In addition, the stimulation parameters (electrode position and size, pulse-width, and amplitude) must be carefully considered when designing a FES application.
2.3.3 Selectivity and Comfort of Electrodes
The combination of the volume conductor and the nerve models is leading to a full understanding of the dynamics that connect the transcutaneous stimulation parameters with the response of the nerves and fibers. This has opened a research line that aims at optimizing the FES applications, which is translated into stimulating muscles with the highest selectivity, while causing the lowest discomfort to the subject. However, reaching both goals simultaneously is not simple. The two main factors that influence selectivity and comfort are the electrode size and the electrode materials. The electrode is usually built by stacking two layers of conductive hydrogel, a conductive substrate (made of fabric or carbon film) and a substrate. This construction provides a distributed current density over the electrode surface, prevents the skin from burning and guarantees a low electrode-substrate-skin impedance in order to excite the minimum number of afferent nerves [7]. Therefore, when designing a FES application, it is much easier to reach the selectivity and comfort specifications by changing the electrode size. The size of the electrodes is usually chosen in function of the stimulation site, depending on the size of the muscle that wants to be contracted and the proximity of the adjacent muscles that want to be avoided. Smaller electrodes produce more selective activations; however, it might be difficult to activate deep muscles as they generate high current densities that could cause discomfort to the subject [13]. The optimal electrode size can be assessed by combining transcutaneous stimulation FE models (that will establish the selectivity) and experiments in subjects (who will characterize the perceived comfort). The electrical field that is created by the electrodes will penetrate the skin with a certain recruitment depth and width, shaped as a lobe in a cross-sectional view of the muscle (See Fig. 5).
Therefore, the selectivity can be defined as proportionally dependent on the Recruitment Depth (RD) and inversely related to the area that is affected by the lobe, i.e., the Mean Profile (MP) [10, 13]:
On the other hand, the estimated comfort can be assessed as a combination of the pain threshold (ThePain) and the current density of the electrode (CD). None of these parameters can be measured directly; therefore, it has been suggested in literature to measure the perceived pain threshold as the average on ten healthy subjects, and to calculate the current density by dividing the amplitude of the stimulation by the area of the electrode [13]:
A recent study in literature [13] simulated the selectivity and comfort for a set of electrode sizes, activation depths, and fat thicknesses (Fig. 6). Results show that both selectivity and estimated comfort are strongly influenced by the three factors, and they all play a role in the muscle response. This study also indicates that smaller electrodes produce more selective muscle activations and tend to cause less discomfort in the subject when the current density is kept constant. However, the estimated comfort reaches a maximum that does not always correspond to the smaller electrode. In fact, for high activation depths and thick fat layers, smaller electrodes can be less comfortable than larger electrodes. The reason for all this is twofold: electrical stimulation is more tolerable when it is felt on a smaller portion of the skin (less receptors are activated), and the current with smaller electrodes (at a specific current density) penetrates less deep into the tissues than with a larger electrode.
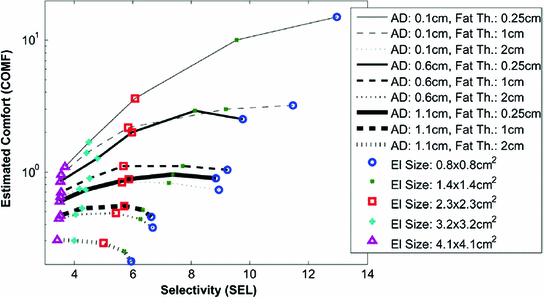
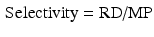
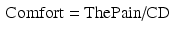
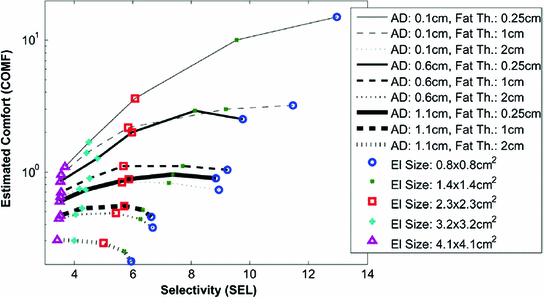
Fig. 6
Comfort versus selectivity, for a various set of Activation Depths (AD), Fat Thicknesses (Fat Th), and Electrode Sizes (El Size) (Reproduced from [11])
3 Upper Limbs—Practical Guide
Upper limbs are involved in a countless amount of activities of daily living (ADL) that include reaching, grasping, and manipulating objects.
This section aims to be a practical introduction to transcutaneous FES on upper limbs, where practical exercises are suggested in order to experimentally prove the basic concepts described in the previous section and check at first hand the main challenges related to transcutaneous FES application in upper limbs.
General notes In order to use transcutaneous functional electrical stimulation in efficient and safe manner it is necessary to obey certain elementary rules.
As explained in the introduction, FES is achieved by applying electrical current to the muscle or nerve fibers through electrodes placed on the skin surface. It is important that the surface of the electrode is conductive, adhesive, and has good contact with skin along the entire surface. Most commercial electrodes used today have a layer of conductive gel which ensures these properties. In any case, before placing the electrodes one should check if the gel is moist and adhesive, as dried up electrode can cause discomfort or pain during stimulation.
When starting the stimulation it is advised to start with the lowest recommended amplitude and gradually increase it until the required response is achieved.
3.1 Upper Arm
Upper arm muscles act mainly at shoulder and elbow joints; therefore, the main functional task carried out by these muscles is the reaching task.
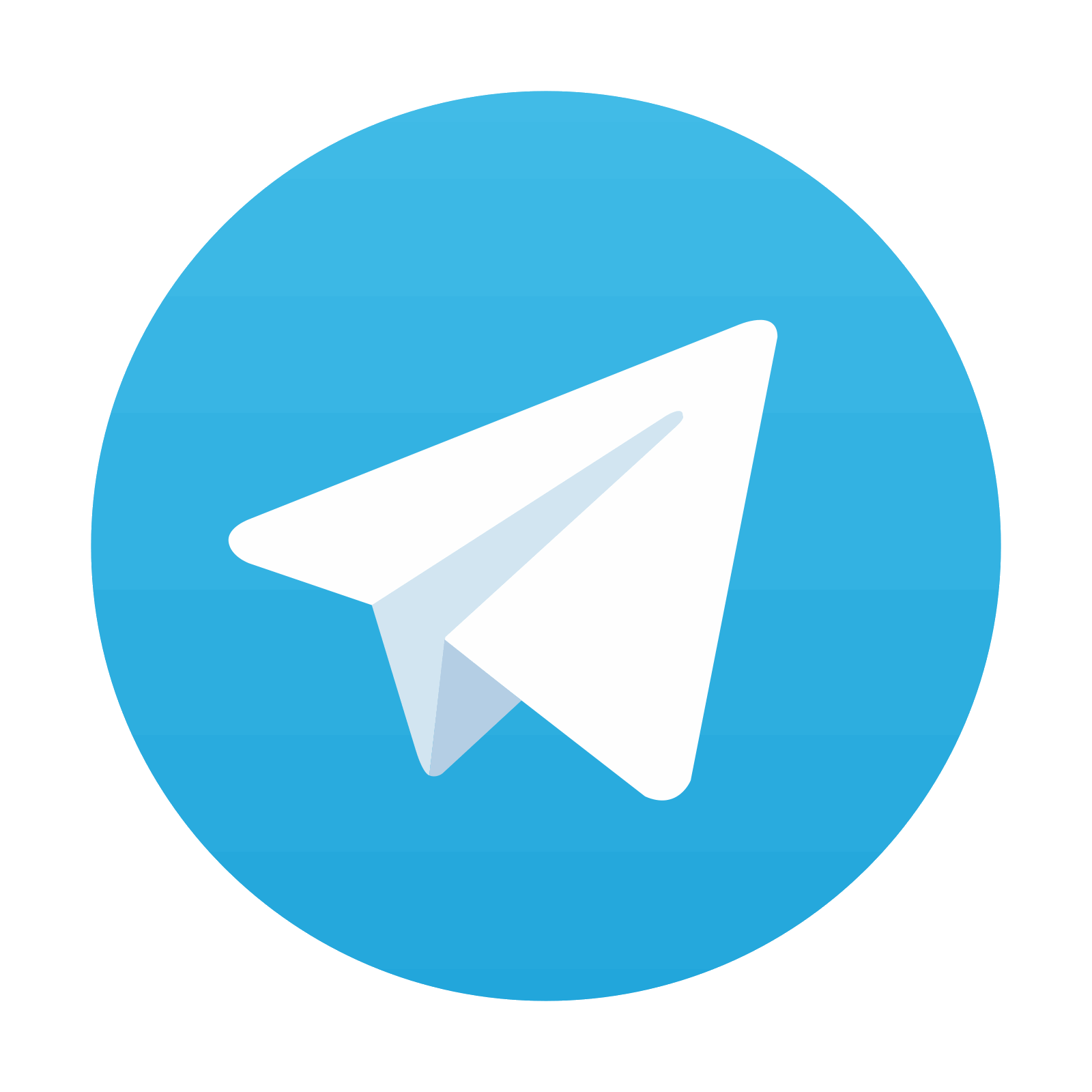
Stay updated, free articles. Join our Telegram channel
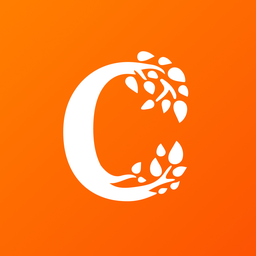
Full access? Get Clinical Tree
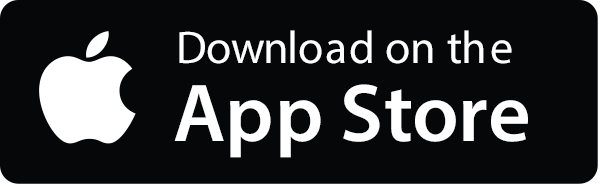
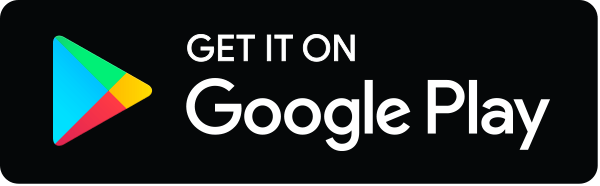