Fig. 1
The translational approach
Organism models are used in the second and third stages. The identification of infectious, traumatic, and genetic events causing a disorder is initially done on patients; then replication of the findings in other species provides confirmation. This has been well illustrated in the field of human reproduction with research into the role of the SRY (sex-determining region of the Y chromosome) gene. Molecular analysis [2, 3] suggested that the SRY gene may be involved in abnormal development, structure, and function of the testes. A paper by Koopman et al. [4] provided definitive evidence on the contribution of Sry 1 to the development of the testes. Transgenic mice with Sry appeared to have testes yet were identified as females with an XX karyotype. The testes were smaller than in wild-type males and the carriers were sterile. The mouse model thus demonstrated that Sry was necessary but not sufficient for the development of functional testes. Deletion of all or part of a gene makes it possible to come closer to the putative causes of the disease. Not only can organism models be used to test hypotheses, but they can also help record the natural history of the disease by elucidating the pathophysiological pathways specific to the disease. While magnetic resonance imaging (MRI), biopsies, and postmortem tissue analyses can provide direct cues to pathophysiological pathways, MRI is not always sufficient, and biopsies cannot be performed for diseases affecting complex organs such as the brain. Organism models are therefore essential at this stage; they help overcome such difficulties as they can be used for histology, biochemistry, and physiology. Organism models can also be used as an indicator for further examinations to be prescribed for patients. Brain imaging is both stressful and expensive. The reporting of pathological signs in the brain of an organism model would be an argument for prescribing MRI examinations. Organism models also contribute to preclinical studies and are essential for assessing any alleviation obtained through treatment and for detecting possible iatrogenic effects [5].
Organism models can overcome difficulties involved in researching rare disorders, including the small number of patients, small family size, the scattering of patients over geographical areas, clinical heterogeneity, and difficulty in collecting biological samples. This is of particular relevance for studying autism as a relatively high prevalence of autistic disorders has been reported in rare diseases (e.g., Smith–Lemli–Opitz syndrome [6–12], Sanfilippo syndrome [13–16], Smith–Magenis syndrome [7, 17–21], Angelman syndrome [22–32], fragile X [33–40], Rett syndrome [41–49], trisomy 21 (Down syndrome) [50–56], etc.).
The organism model approach must not be seen outside the evolutionary context of phylogenesis as it is based on the principle that human and nonhuman species had common ancestors, which means common causes of impairments with an impact on common functions and common biological processes are found in both human patients and the model.
While organism models make a major contribution to the different stages needed to identify strategies for treatment and/or alleviation, they are not always indispensable. We must remember that the first cure for a genetic disorder—phenylketonuria—was a low-phenylalanine diet [57], and, ironically, the diet was found without studying any organism models and was adopted directly for human patients.
2 Four Criteria for a Causal Model of Disease
The need to find organism models in a translational perspective first led to the selection similarities between human and other species. A species or strain was considered to model a disease when the observed characteristics of the organism tallied with anatomical, physiological, or pathological criteria defining the disease. Phenomenological similarities were considered to be satisfactory, but the causes of a given pathology, the intermediate pathways between the cause of the disease and the pathological manifestation, were not necessarily the same for the model and humans. Certain strains of laboratory mice display voluntary alcohol consumption, but the reasons for drinking are probably not the same for C57BL/6J mice as they are for inveterate drinkers.
McKinney [58] and later Robbins and Sahakian [59] proposed rules to improve the validity of organism models, but with the development of molecular tools after 1980 came more stringent criteria [60]. Our proposal here is that a causal model should meet four criteria: (1) the disorder must have identical etiology in humans and the organism model and therefore (2) a similar molecular signature; (3) the organism model and humans must have comparable pathophysiological pathways; and (4) experimental analysis of the model must match clinical observations.
2.1 First Criterion: Identical Etiology
The possibility of generating genetically modified organisms provided an opportunity for creating models where the cause of the disease was expected to be the same as in human patients. The first models used the possibility of selecting the abnormal human allelic form that is associated with a given disease and overexpressing it in an organism model. Transgenic and knockout organisms now offer possibilities for generating ad hoc models by overexpressing or deleting the whole gene. Silva et al. [61, 62] published articles on the first study targeting a gene contributing to brain function and demonstrated that mice lacking the alpha-calcium–calmodulin-dependent kinase II (alpha-CaMKII) gene displayed impaired long-term potentiation (i.e., poor synaptic plasticity) and correlatively impaired spatial learning. The deletion of a gene in an organism model may be all that is needed to create the model, as shown in the case of fragile X syndrome. Oberlé et al. [63] and Yu et al. [64] simultaneously reported that the syndrome was the consequence of both the instability of a DNA segment and abnormal methylation. Both repeats and hypermethylation shut down the transcription of FMR1 with a loss of the FMR protein that contributes to synaptic functions. The two genetic events create a loss of function similar to the one generated by gene targeting. A homologous Fmr1 knockout mouse model was developed [65]. FMR knockout mice present various cognitive disorders and brain dysfunctions similar to those generally observed in persons with fragile X syndrome.
The early targeting technology that proceeded by deletion of the whole gene or collective deletion of several genes was limited by the possible presence of regulatory sequences on the noncoding regions of the genome. Noncoding regions carry microRNA (miRNA) sequences that regulate transcription factors. Thousands of miRNAs have been reported in mammals [66]; miRNAs contribute to the development of diseases [67] and also to neuronal and cognitive development [68]. The deletion of the full gene (intronic plus exonic sequences) cumulates the effect of the protein for which the gene codes and the effect of other proteins may be regulated by the miRNAs. With more recent models, it is possible to avoid the pitfall of the whole deletion. Deleting crucial exons or preventing transcription can overcome the difficulty arising with the presence of regulating sequences.
These sophisticated models help improve the etiological similarity between the organism model and humans; they are moreover of particular interest when a gene may have a number of mutations, each associated with a different disorder. Examining the structure of the lamin A/C (LMNA) gene illustrates the remark. More than 200 diseases are associated with the numerous reported mutations. Table 1 indicates the different mouse models that have been developed.
Table 1
Mouse model of lamin A diseases
Model name Reference: first description model | Construction | Phenotype (tissue highly affected) |
---|---|---|
Lmna K.O [185] | KO | |
Gene trap | ||
KI-L530P | Premature aging | |
KI-H222P | Muscular dystrophy and dilated cardiomyopathy | |
Transgenic human lamin AM371K-Flag | Increased eosinophilia and fragmentation of cardiomyofibrils, nuclear pyknosis, and edema | |
KI-N195K | DCM-CD1 | |
KI-intron 10—150 bp exon 11 deleted—intron 11 deleted | Premature aging (bone and adipose tissues, body weight …) | |
Transgenic human BAC 164 kb | Premature aging (vascular system) | |
Transgenic human complete coding region of lamin A, including exons 1–11, intron 11, and exon 12 | Premature aging (skin) | |
Transgenic human minigene (cDNA)-Flag | No phenotype | |
LCO [195] | KI-intron 11—150 bp exon 11—intron 11 deleted | No phenotype |
KI on HG model: CSIM mutated in SSIM | Premature aging | |
KI on HG model: CSIM mutated in CSIL | Premature aging | |
KI-intron 10 deleted—exon 11 present—intron 11 deleted | No phenotype | |
KI on LmnaPLAO: CSIM mutated in SSIM | No phenotype | |
KI-Stop at the second ZMPSTE24 cleavage site | No phenotype | |
Dhe [200] | Spontaneous mutation | Bone density reduced and craniofacial abnormalities |
KI on HG model: CSIM mutated in CSM | No phenotype | |
LAKI model [70] | KI-G609G | Premature aging vascular system and bone density reduction |
Lmna R482Q [201] | KI-R482Q | FPLD (adipose tissue) |
Lmna ∆K32 [202] | KI-DelK32 | Striated muscle maturation delay and severe metabolic defects |
KI-R298C | No obvious phenotype but molecular defects in peripheral nerve tissue |
The Hutchinson–Gilford progeria syndrome is caused by a de novo point mutation (coding nucleotide 1824: c.1824C>T) causing an altered splicing in the gene’s pre-mRNAs. The mutation leads to the elimination of the 3′ half of exon 11 (150 bp encoding 50 amino acids) resulting in a truncated and toxic form of prelamin A called progerin [69]. The relevant mouse model for Hutchinson–Gilford progeria [70] carries the equivalent exon 11 point mutation in the homologous mouse Lmna gene. The genes associated with ASD present a similar complexity. The neuroligin 4 gene encompasses 816 amino acids. A point mutation (coding nucleotide 396: 396 G>T) transforms GAC into a stop codon TGA, resulting in a truncated protein [71], whereas the deletion of two base pairs in codon nucleotide 418 causes a premature stop codon in nucleotide 429 causing a truncated protein [72]. The two proteins do not have the same truncation and different protein domains are affected. The second mutation is associated with intellectual deficiency and the first with ASD, independently of cognitive abilities since 396 G>T is present in Kanner and Asperger ASD [73]. Thirty-seven mutations are known for their association with diseases (Weizmann Institute of Science; http://www.genecards.org/). Targeting a gene associated with a disease is not enough. Recent advances in molecular genetics provide tools able to mimic more accurately the etiology of the disease.
Several techniques are available for modeling complex disorders as chromosomal syndromes. For half a century now, we have known that trisomy 21 (Down syndrome) is caused by an extra copy of chromosome 21 (HSA21), but we still have no knowledge of the function of HSA21 genes in the traits characterizing the syndrome. The estimated number of genes encompassed by HSA21 is relatively small (approximately 300), thus making it feasible to adopt a genotype–phenotype correlation approach for HSA21 genes and the cognitive characteristics observed in TRS21 [74, 75]. A region between D21S17 and ETS2 has been reported as being associated with most of the Jackson signs, including cognitive deficiency [76, 77]. Smith et al. [78–80] developed a mouse model of trisomy in which extra fragments from the human D21S17–ETS2 region were inserted into the mouse genome; the D21S17–ETS2 regions being syntenic to MMU16, they thus created segmental trisomy for the region. As an extra copy of a chromosomal fragment including the Dyrk1a gene was known to generate cognitive disorders, Altafaj et al. [81] developed a transgenic mouse overexpressing the Dyrk1a gene only, as it was suspected of playing a major role in cognitive disorders. Methods such as mutagenic insertion and chromosome engineering resource (MICER) [82] or targeted meiotic recombination (TAMERE) [83] offer opportunities for developing mouse models of partial trisomies or partial monosomies. MICER has been widely used to generate segmental trisomies in Down syndrome studies and could be of interest for generating mouse models of 2q37 deletion [84–88], 15q11-q13 duplication [89–92], 16p11.2 duplication [93–97], 16p11 deletion [98–102], and 22q13 deletion [35, 103–112] which have all been reported to be associated with autistic traits.
Generating an organism model produces difficulties that are organism dependent and/or gene dependent. No one knows why one extra copy produces a dramatic phenotype in humans and a mild phenotype in the mouse or, even more striking, why a number of genes in the D21S17–ETS2 region are not reactive to chromosomal extra copy (Roubertoux et al., submitted).
2.2 Second Criterion: Similar Molecular Signatures
The protein is the final stage in a series of transitional steps from transcription to translation. Each step can be considered as an integrative process, whereby the generation of information at one level relies on information provided by previous elementary steps. The price for this generation of information is weaker causality of the link from DNA to protein in a given cell, with several immediate consequences for modeling diseases. Identical etiology is not sufficient as the same trigger may produce different effects in two species. The mouse is the most widely used organism for modeling human diseases, but a mouse is not a human. The Mouse Genome Sequencing Consortium found that the ratio of non-synonymous mutations (changing the amino acid and protein) to synonymous mutations (not affecting the amino acid and protein) in the mouse and the human is 0.115, indicating a weak but not negligible dissimilarity of mouse and human proteins [113]. It is also important to note that the study of tissue-dependent cross-species expression differences provides a caveat for the development of organism models. Investigations of the neocortex with a large pool of orthologous genes found that 5 % of genes were expressed differently between humans and 21 % between humans and mice [114]. Co-expression of several genes is also important as gene interactions, cascades, or additive effects may contribute to the disease. While some studies have shown the network pattern of transcripts to be well preserved across the species, patterns of co-expressed genes associated with a disease can differ across the species [115].
While all species have a common ancestor, not all species are suitable to model a disease. Subtle changes in the structure of a protein may disqualify a species; for example, a change to p.Ser658Pro in the SEL1L gene is pathogenic in the dog and could be a link in canine progressive early-onset cerebellum ataxia. SEL1L is a transmembrane glycoprotein in the endoplasmic reticulum. The p.Ser658Pro amino acid change is positioned in one of several Sel1-repeat motifs of the protein. The Ser658 sequence is conserved in all aligned vertebrates, insects, and the hemichordate acorn worm, but only the orthologs of C. elegans and of yeast differed at that position [116] disqualifying the two last species as model.
Metabolic differences may complicate the modeling of a disease. Lesch–Nyhan syndrome in mice has stimulated the interest of psychiatrists considering clinical similarities with autism (self-injury and cognitive impairment) [60]. Lesch–Nyhan syndrome is an X-linked recessive disease due to a mutation in the hypoxanthine phosphoribosyltransferase (HPRT) gene. Several mutations alter or modulate HPRT production, and patients with the syndrome in fact have no levels or only residual levels of HPRT. This aberrant metabolism is associated with cognitive disorders and self-injury in the Lesch–Nyhan syndrome. Invalidation of the HPRT gene present in mice should have provided an excellent model of mouse Lesch–Nyhan syndrome [117, 118], but, unfortunately, none of the mice lacking HPRT displayed self-injurious behavior. An explanation for this was found in a metabolic difference between mice and humans. Nonmutant mice did not salvage circulating hypoxanthine, suggesting that mice are protected against HPRT loss and that purine metabolism is less HPRT dependent in mice than in humans. Wu and Melton [119] examined adenine phosphoribosyltransferase (APRT), the second enzyme involved in the purine salvage pathway, observing first that at different ages HPRT/APRT was lower in mice than in humans. They then administered an inhibitor of APRT (9-ethyladenine) to mice lacking HPRT that did not display self-injurious behavior. These HPRT −/− mice, when given the inhibitor, recorded a high frequency of self-injurious behavior. Here, the genes involved in the disease are orthologous; their molecular signatures are similar, but the HPRT/APRT balance is not the same in the mouse as it is in humans. APRT compensates for the lack of HPRT in the mouse but not in humans.
Differences in characteristics in the model and in the human make it impossible to develop a model. The mouse has most of the immunological features found in humans but does not have FcαRI, FcγRIIA, granulysin, or caspase 10, and the human species does not have GlyCAM; and the serum IgA is mostly polymeric in the mouse and mostly monomeric in humans. These differences therefore disqualify the mouse as a model for diseases involving these. Other species such as Drosophila [120] or zebrafish [121] can provide alternatives for the study of innate immunity-related diseases. Adjustments are needed for less challenging differences such as the effect of IL-7R deficiency which blocks both B- and T-cell development in mice but only blocks T-cell development in humans.
The identification of cellular mechanisms has brought prospects with cellular models, but the model developed is more a model of the cellular or metabolic conditions required for the onset of the disease rather than a model of the disease. Several models exist for neurological and developmental diseases (Caenorhabditis elegans, zebrafish, Drosophila, and yeast). Mason and Giorgini [122] reviewed the yeast cell model used to test for a number of mechanistic relationships between the abnormal expansion of a polyglutamine tract and huntingtin protein toxicity. Tauber et al. [123] published the complex gene expression profiling in mutant yeast for huntingtin, and the resulting chart has been crucial for the analysis of the transcriptional consequences of huntingtin toxicity.
The selection of an organism model therefore requires three preliminary tasks. The first is to document all the biochemical aspects of the disease, collecting the biological parameters in different patients and, when possible, establishing developmental data. The second is to choose the organism model considering the similarity of its typical molecular signature to the human signature. The last step is to compare molecular signature deterioration in patients and in models after induction of the pathological modification.
2.3 Third Criteria: Comparable Pathophysiological Pathways
Pathophysiology, the molecular indicators, and clinical observations are part of the diagnosis itself. Pathophysiological measurements can be used to monitor the development of the disease and/or its reaction to treatment. The organism model chosen must therefore have anatomical and physiological properties matching those observed in human patients. The pathophysiological parameters in both humans and the organism model should be assessed using the same techniques, e.g., magnetic resonance imaging (MRI) and transcriptional microarrays, so as to minimize disparities.
2.3.1 Sensorial and Motor Nervous System
Modeling is not direct as the comparison of a human patient and a model is not linear. Humans diverged from the common ancestor shared with apes, mice, and zebrafish approximately 5 million, 70 million, and 100 million years ago, respectively; and despite the common origin, they have developmental, anatomical, and physiological differences. Some differences will have only a minor impact on modeling. The sensorial development of mice and humans has similar timing, with immaturity at birth including late visual development, a long poikilothermic period (1 week in mice and 1 year in humans), and early tactile, gustatory, and geo-sensitivity [124]. Discrepancies appear with hearing which, in humans, functions prenatally at around 27 gestational weeks but not until 1 week after birth in mice [124]. Olfactory bulb organization is not the same, with a difference between humans and most mammals which have two olfactory systems: the main one helping detect dietary signals and the accessory olfactory system detecting socially related cues. The accessory system is well developed in rodents, mediated by the Jacobson’s organ, but is only vestigial in humans. The main olfactory system in rodents has a zone needed to detect innate aversive odors and the system is much more plastic than in humans where there is no such zone [125].
Greater differences are seen in the anatomy of the nervous system in rodents and in primates. The medullary organization of motoneurons and, in correlation, the effects on locomotion and dexterity differ. The corticospinal tract is a descending medullary pathway and dexterity depends on it being intact. In rodents, damage to the corticospinal tract will not disrupt locomotor activity and any forepaw alteration is recovered, whereas in nonhuman primates, locomotor activity is permanent and hand dexterity is only partially recovered. Damage to the corticospinal tract in humans is even more severe and less reversible. Organization of the tract differs across the species and is consequently a factor in the selection of an organism model for studying motor disorders. The number of fibers varies, the estimated number being greater in humans (1,101,000) than in nonhuman primates (400,000) and rodents (137,000). Direct corticospinal connections are found in motoneurons in primates, including humans, but in rodents there are no direct connections between the corticospinal neurons and the cervical motoneurons innervating the limb muscles. The organization of the fibers in the spinal cord also differs. A large percentage of corticospinal fibers follows an ipsilateral descending medullary pathway; in rodents most of the fibers are in the dorsal column, whereas in primates most are in the lateral column [126]. The anatomical and physiological organizations of descending medullary pathways in rodents are limiting factors to model medulla stroke and therapeutic assays.
2.3.2 Brain Functions
The most difficult challenge is to model complex organization such as the brain. While the prefrontal cortex does exist in rodents, it shows less differentiation than in primates; the cortical layers differ, with the mouse that has a “smooth brain,” disqualifying the species as a model of lissencephaly. The brain is a set of 52 areas defined by specific cytoarchitectony and function [127]. Area anatomy is species specific and differs dramatically across mammals, but it is possible to find highly preserved anatomical properties or functions in an area. The prefrontal cortex in rodents shows connections that are more similar to primate median cortex than to primate prefrontal cortex connections [128–132].
The neurotransmission system does not appear to be differentiated across mammal species, including rodents which have been used for a long time to determine the functioning of neurotransmitter systems, but some receptors may have specific properties. The 5-HT6 receptors in the mouse, rat, and human have strong sequence homology, but the pharmacological properties of the mouse receptor differ from the rat and human receptors. The mouse 5-HT6 receptor does not have the same affinity for the serotoninergic agonists and antagonists as the rat or human 5-HT6 receptor, the difference being due to a binding pocket in the mouse [133]. Great precaution should be exercised before modeling diseases involving the 5-HT6 receptor, and investigations are needed before generalizing the results to other serotoninergic receptors and to other neurotransmitter systems.
Incomplete similarity of properties of a structure in humans and an organism model is not sufficient justification for rejecting the organism as a model. Similarity exists on a number of scales and not all are relevant for comparison. The simplest is not necessarily the worst. Size or weight may be quite simply sufficient. The methyl-CpG-binding protein 2 (MECP2) gene is involved in Rett syndrome. Mecp2-null mice present brain reduction [134] that is also present in patients with Rett diagnosis [135]. The small size of brain structures in persons with trisomy 21 is well documented [136]. This small size provides an excellent framework to model small brain structures in mouse models of trisomy 21. Surprisingly, the smallest organisms may provide unexpected models that push back the limits for modeling the pathophysiological pathways. Mushroom bodies of Drosophila are homologues of the mammalian hippocampus [137]. The mutations producing small mushroom body size also produce a small hippocampus. This anatomical homology offers scope for modeling the pathological effects triggered by the minibrain gene that is an ortholog of DYRK-1A. Minibrain mutation reduces the size of mushroom bodies, as does a mutation of DYRK-1A that is located on the D21S17–ETS2 chromosomal region of chromosome 21, and contributes to the trisomy 21 phenotype [138, 139].
Not all properties are useful for modeling the effects of a disease on the physiological pathways. The relevant property or dimension should first be determined and selected. Any pathophysiological criterion thought to be similar must be examined on an ad hoc basis in the organism and for measurement being performed across the species. Different techniques have been proposed [140]. The Structural Difference Method correlates the anatomy of the human species with other species by using the Foundational Model of Anatomy [141–143] and this could be extended beyond the anatomical field.
2.4 Similar Clinical Characteristics
The main benefit expected from reproducing clinical characteristics in models is a global validation of a beforehand approach and the determination of immediate markers for therapeutic follow-up. A holistic approach may be weighted against an analytical approach or a one-species strategy against a multiple-species strategy. The clinical characteristics of the disease can be out of the organism repertory suggesting to model the reproduction of the conditions that permit the coming out of the characteristics rather than the characteristics themselves.
2.4.1 Holistic Versus Analytical Approach
Some diseases are characterized by a main feature, e.g., achondroplasia. In most cases, several features are present, e.g., Marfan syndrome (eyes, skeleton, and cardiovascular system are impacted) or Rubinstein–Taybi syndrome (nervous system tumors, intellectual deficiency, and finger deformity). More complicated cases are trisomy 21 with 25 characteristic traits [144] and Williams–Beuren syndrome with a complex pattern of physical, physiological, and cognitive traits [145–148]. A choice then has to be made necessary to select a holistic model reproducing the full pattern of impaired features or an analytical model miming only one of the salient features of the disease.
The holistic model is tempting but it has been rarely employed with success. Difficulty in spatial learning goes together with ability in associative learning in persons with trisomy 21 [149]. The difficulty and the ability are found in most of segmental models of trisomy 21 [136, 150], but the higher incidence of leukemia observed in human patients is not found in trisomy mouse models. Giving priority to a holistic model at any price could result in conceptual and experimental permissiveness.
The holistic model may be initially perilous as the disease affects several tissues and the degree of homology is not the same for all tissues. It would be unrealistic to attempt to find tissues modeling the human prefrontal cortex in the fly or even the mouse. There is, however, partial homology shared by the hippocampus and Drosophila mushroom bodies as their size depends on the mutation of an orthologous gene as described above [137]. It is possible to model hippocampus but not prefrontal cortex dysfunction in mice and flies making it impossible to develop a holistic model of cognitive disorders in only one species.
The holistic model does not tally always with the clinical observation. While several authors attempted desperately to develop a holistic model of schizophrenia in rodents, [151] limited the attempt to the measure of attention because attention defect appears as obvious in schizophrenic disorders. The three scales of the ADI-R (Autism Diagnostic Interview–Revised) share few genetic covariances [152] and different Genome Wide Association Studies (GWAS) correlates [153]. These results exclude a holistic modeling of autism.
2.4.2 When Clinical Features Are Not Found in the Repertory of the Organism Model
Certain characteristics of some diseases cannot be found in organism models as the anomaly concerns human-specific fingerprints. A way around this difficulty can often be found by adapting the task to the repertory of the species. The facial features of persons with trisomy 21 cannot be modeled in the mouse, but Reeves group [154] suggested considering differences between trisomic and euploid mice for the facial bone junction to model trisomic appearance. Left or right paw preferences are not stable in the mouse, making it difficult to develop models for laterality anomalies associated with neurological disorders. Some mice are well lateralized (left or right) while others are ambidextrous [155]. Well-lateralized mice are considered to be the equivalent of right-handed persons, and ambidextrous mice of left-handed persons. This then provides the basis for defining a direction continuum (from well lateralized to ambidextrous) that can be used to model direction of laterality. Lissencephaly in humans can be detected by noninvasive techniques, but the mouse and most rodents have a smooth brain disqualifying them as models for lissencephaly. The solution here is to consider defective neuronal migration as the cause of the disease. As lissencephaly is triggered by a mutation of the PAFAH1B1 (platelet-activating factor acetylhydrolase, isoform 1b, subunit 1) gene, it is possible to model the defective neuronal migration as proposed [156].
Inability to communicate and language impairment are major features in intellectual deficiency and autism and remain as stumbling blocks for the organism modeling strategy. Several authors have considered vocalization in mice as an indicator of communication [73, 157–159]. The solution offered by the studies on lissencephaly should be adapted to language. The correlation between the scores in communication and language impairment scale of ADI-R and the transcript clustering suggests to model the transcript clustering.
3 Top-Down Analysis: Analyze the Modeled Clinical Trait but Also the Physiological Correlates
A major benefit of an organism model can be in uncovering the links between primary causes and clinical manifestations by entering observations into a series of causal imputations. Genetic disease is caused by an event producing an abnormal protein or lack of protein in an organ. An organism model can be used to track the protein deficiency through to the cellular compartment where it triggers the dysfunction(s). The task becomes difficult when having to analyze visceral organs or the heart, and the greatest difficulty is encountered with the brain. We know from Brodmann [127] that the brain is not an organ but rather a set of interacting structures, and these structures may react differently to a protein deficiency and also to protein replacement. Gene product exerts differential modulation even on closely related brain structures. Selective inactivation of the tyrosine hydroxylase gene in neurons prevents dopamine synthesis and triggers mouse behavioral abnormalities including hypophagy, hypoactivity, lack of nest building, and disinterest in a palatable diet. Recovery of dopamine production in every structure of the ventral striatum did not induce similar behavioral rescue but restored feeding on regular chow, nest-building behavior within the caudate putamen, and exploratory behavior within the nucleus accumbens; the preference for sucrose was recovered by restoring dopamine to one or both of these regions [160]. The gene targeting or overexpression strategy operates before the first meiosis, and all the cells in the organism then carry the genetic modification preventing so the identification of the cellular target of the gene. The identification of the cellular target of the gene requires other approaches.
3.1 The Tissue-Specific Strategy
With the tissue-specific strategy, the deletion or overexpression can be restricted to a group of cells. The deletion of a gene may not produce a phenotype when the deletion is expressed in the hippocampus, cortex, or striatum, the impairments appearing only when the protein is lacking in the cerebellum. The conditional approach leads to the discovery of the tissue targeted by the disease. The structure—the group of cells, cell, or even the cellular compartment where the gene is targeted—can be identified after successive elimination is achieved when using the new conditional approaches. Several strategies are now available for generating tissue-specific targeted genes and even brain tissue targeted genes. The most widely used is the Cre–loxP system in which the reporter is selected to drive the recombinase toward an identified brain region. Madisen et al. [161, 162] published a list of Cre reporters identified as being expressed in brain regions (cortex, hippocampus, striatum) or in more specific tissues (parvalbumin-positive interneurons, cholinergic neurons, dopamine neurons). Song et al. [163] observed the specificity of Cre reporters for certain regions of the hypothalamus. Tissue-specific gene targeting removes the main obstacle to identifying neuronal networks. Taniguchi et al. [164] overcame the difficulty by identifying 17 Cre reporters of differing degrees of specificity to GABAergic neurons in the mouse. Gene targeting in serotoninergic and in dopaminergic neurons [165] offers the possibility of modeling psychiatric disorders associated with dopaminergic impairment, for schizophrenia or with rare genetic disease—Angelman [166–168] or with serotonin [169].
The best solution is to give up the holistic model and to develop analytical models, each being tested in the most suitable species. Selecting the species that has the strongest homology for one of the impacted tissue and another species that has the strongest homology for another tissue is the best solution. This is expensive and time consuming.
These different approaches show the trend in developments with the new generation of organism models. The top-down approach includes the different levels of functioning, starting with the genes and ending with observable manifestations. All four criteria are met, generating a top-down approach able to include the genetic, cellular, physiological, and behavioral levels in a causal string of imputations.
3.2 Transversal Approach and the One-Tissue/One-Species Strategy
The transversal approach consists in reproducing the top-down approach in at least two species. Finding the same causal string in several species reinforces the confidence in the reliability of the models.
One example is the investigation of defects of the enteric nervous system in Hirschsprung disease [170] that have been analyzed in mice [171, 172], zebrafish [173, 174], and C. elegans [175]. Brain disorders are also good illustration of the power of a transversal approach. Angelman disease has been associated with maternal deletion at 15q11-q13, resulting in a paternal uniparental disomy. The disruption of the function of the maternally inherited UBE3A has now been well established [176]. A decrease in dendritic arborescence has been reported in postmortem studies; more specifically, the mutation has been associated with a reduction in dendritic spines in pyramidal neurons of the visual cortex [177]. The association with UBE3A maternal deficiency (m−/p+) has been reproduced in the mouse, causing reduced spine density of basal dendrites in pyramidal neurons of the visual cortex [178]. UBE3A maternal-deficient mice expressing a Ube3a YFP fusion gene display abnormalities in dendritic spine morphology, number and length in cerebellar Purkinje cells, and pyramidal neurons in the hippocampus and cortex [23]. A similar result was found with Drosophila. Reduced dendritic branching of sensory neurons appeared as the consequence of breeding a UBE3A-null mutant [179]. Studies have confirmed the functional link between the gene and the dendritic shape and number across the species. Studies of Rett syndrome extended the list of organism models for human neuron abnormalities to mice, flies, and batrachians. Postmortem brain analyses have shown that patients diagnosed with Rett syndrome have a smaller number of dendrites [180]. The same result was found in the mouse model of MECP2 disorders. Mecp2 mutant mice (Mecp2 tm1.1Jae /Mmcd mice) were found to have reductions of neural cell size [181], dendritic branching, and spine density in layer 5 motor cortical neurons [182]. The dysfunction of MECP2 in Drosophila induces motoneuron dendritic defects characterized by a reduction of the number of dendrites [183]. Reduced dendritic formation and abnormal morphology and connectivity are obtained with a Xenopus model, with MeCP2 dysfunctions [184].
The possibility of modeling a disease in an organism depends on the possibility of reproducing the link between the cause and the effect and is limited by the biological similarity of the species.
The transversal approach does not always fit the properties of the organism models, although most animals, and in particular most mammals, have similar physiological functions. We noted above a certain number of caveats in the field of neurotransmission and specifically for differences with the 5-hydroxytryptamine-6 receptor in humans and mice. We suggested that the focus should be on the endophenotypes or traits not found with the most commonly used models. Sometimes a species considered as exotic can present an unexpected similarity with humans; this is the consequence of non-teleonomic evolutionary processes whereby the characteristics of one taxon are included in another taxon. It is generally believed that the most suitable organism models are primate models, but this is not always the case, and phylogenetic proximity is no guarantee of the proximity for all characteristics. Cortex folding varies within the phylogenetic classes including the primate order. Humans have highly convoluted brain, there are fewer convolutions in the rhesus monkey, and none at all in the lissencephalic marmoset. There is no folding of the cortex in rodents, with the exception of the South American rodent capybara. Surprisingly, the best model for studying pathologies associated with brain folding patterns is the ferret which, like the human species, has divergent development of radial fibers resulting in a folded cortex.
As a general rule, there is no single species that can be used as a universal model for rare diseases. Each disease requires an organism model; and no single species is the right model for any one disease as each trait characterizing the disease may require a separate organism model. The one-species strategy has prevailed for financial reasons and because it is difficult to set up research teams in one laboratory with experience in keeping different species and expertise in the physiology and ethology of the species.
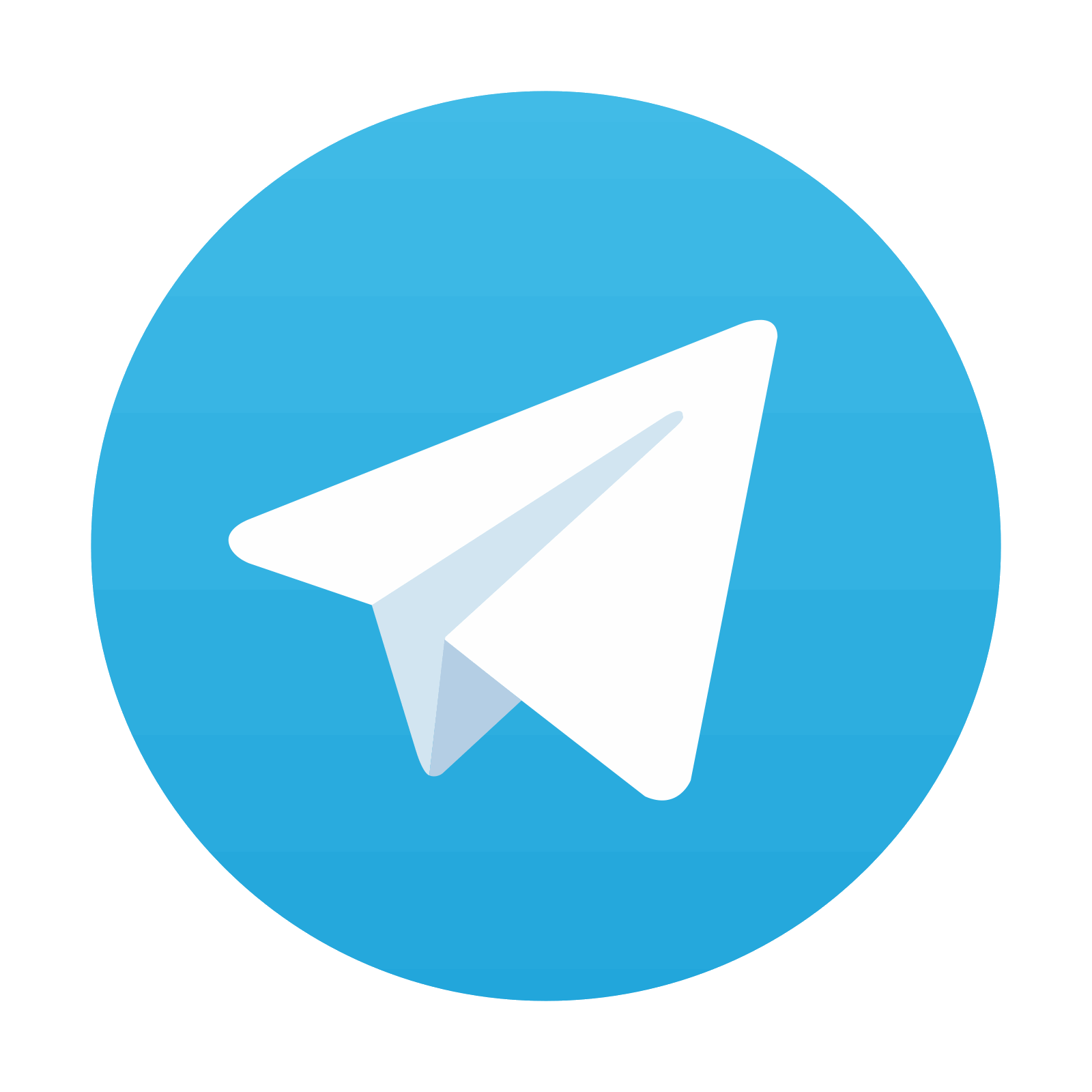
Stay updated, free articles. Join our Telegram channel
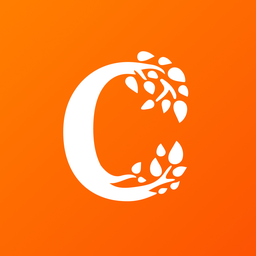
Full access? Get Clinical Tree
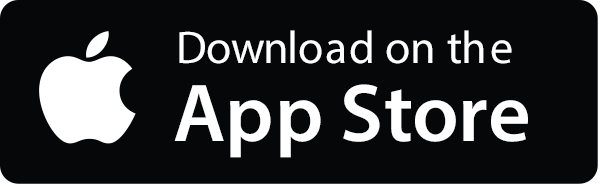
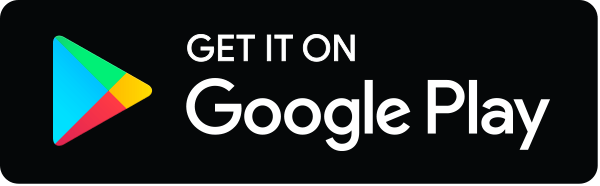