CLINICAL CASE | Alzheimer Disease
A 79-year-old man has become forgetful, often misplacing items at home, and sometimes is confused when paying for his groceries. His family reports that his forgetfulness seems to be getting worse. On neurological examination, he reports the correct date and knows where he is and why he is there; he has normal speech. However, he is unable to recall the names of three unrelated words 5 minutes after correctly repeating them. When asked to perform simple addition and subtraction, he is slow and has difficulty. His mental status was further evaluated, which revealed additional cognitive impairment. He was diagnosed with Alzheimer disease, based on his neuro-psychiatric examinations and brain imaging studies.
Figure 1–1 shows, side by side, a photograph of a brain from a person that had Alzheimer disease (A1) and a normal brain (B1). Magnetic resonance images (MRIs) are presented below (A2–5; B2–5). The appearance of brain slices will be explored further, beginning with Chapter 2, as we learn about the brain’s internal structure. But we can take this opportunity to consider changes to the cortex and ventricular system as revealed on slices of the living brain. Parts 2–4 present a series of MRIs close to the transverse plane (see inset; also, Figures 1–16 and 1–17). On these images, white and gray matter appear as different shades of gray and cerebrospinal fluid, black. Cranial fatty substances (for example, skin and in the bony orbits) are white. Note how the ventricles are thin in the healthy brain (right column), but dilated in the diseased brain (left column).
The hippocampal formation (Figure 1-10A; see Chapter 16) also becomes atrophic in Alzheimer disease. This is seen in the coronal MRIs in Figure 1–1. The generalized cortical atrophy and ventricular enlargement are also apparent on the transverse MRI.
You should try to answer the following questions based on your reading of the chapter and inspection of the images. Note that the description of key neurological signs that follow the questions also will provide the answers.
1. Why is the ventricular system affected, even though it is a non-neuronal structure?
2. Are some brain areas more severely affected than others?
Key neurological signs and corresponding damaged brain structures Brain of person with Alzheimer disease and healthy brainNo description is necessary; the amount and extent of cortical atrophy is obvious in the brain of the person that had Alzheimer disease (A1). Cortical atrophy is accompanied by atrophy in subcortical structures as well. Because the volume of the skull is fixed, as brain tissues decrease in volume, there is a corresponding increase in ventricular volume. Thus, ventricular enlargement is a consequence of loss of neural tissue.
Magnetic resonance imagesBoth the generalized cortical atrophy and ventricular enlargement can be seen on magnetic resonance images (MRIs) of the brain. A superior-to-inferior sequence of three images in the transverse plan (see insets) is shown. The MRI in part 2 slices through the anterior horn and atrium of the lateral ventricles, where enlargement is enormous. Because of the extensive cortical atrophy, the cortical sulci are wider and filled with more cerebral spinal fluid. Note the region around lateral sulcus and insular cortex (Figure 1–1A2), where the mixture of a greater amount of cerebrospinal fluid and thinned cortex produces a large dark region. The inset in Figure 1–11A illustrates the insular cortex. The hippocampal formation is key to consolidation of short-term to long-term memory (see Chapter 16). Its reduction in Alzheimer disease, together with degeneration of temporal lobe cortex, leaves a gaping hole in the temporal lobe. Hippocampal degeneration can explain why the patient has poor word recall. These images also reveal that the brain stem is not grossly affected. Although not visible on these images, a small nucleus on the inferior brain surface, the basal nucleus, is severely affected early in Alzheimer disease. This nucleus contains neurons that use acetylcholine. Since these neurons project widely throughout the cortex, with their loss many cortical neurons are deprived of a strong excitatory input. This, together with the gross degeneration, helps to explain the cognitive impairments in the patient. The sizes of the midbrain (parts 3 and 4) and pons (part 5) appear normal.
ReferenceBrust, JCM. The Practice of Neural Science. New York, NY: McGraw-Hill; 2000.
FIGURE 1–1
Brain (top) and MRIs (transverse plane, 2-4; coronal plane, 5) from a person with Alzheimer disease (A) and a healthy person (B). The brain views show generalized atrophy in Alzheimer disease. The MRIs (2-5) show cortical atrophy and ventricular enlargement. The MRIs are T1 images; brain tissues are shades of gray and cerebrospinal fluid, black. (A1, Image courtesy of Dr. Mony J de Leon [NYU School of Medicine], Dr. Jerzy Wegiel [Institute for Basic Research], and Dr. Thomas Wisniewski [NYU School of Medicine]; NIH Alzheimer’s Disease Center P30 AG08051. A2, A3, A4, Images reproduced with permission from Dr. Frank Galliard, Radiopaedia. com. A5, Image courtesy of The Dementia Research Center, UCL Institute of Neurology.)

The human nervous system carries out an enormous number of functions by means of many subdivisions. Indeed, the brain’s complexity has traditionally made the study of neuroanatomy a demanding task. This task can be greatly simplified by approaching the study of the nervous system from the dual perspectives of its regional and functional anatomy. Regional neuroanatomy examines the spatial relations between brain structures within a portion of the nervous system. Regional neuroanatomy defines the major brain divisions as well as local, neighborhood relationships within the divisions. In contrast, functional neuroanatomy examines those parts of the nervous system that work together to accomplish a particular task, for example, visual perception. Functional systems are formed by specific neural connections within and between regions of the nervous system; connections that form complex neural circuits. A goal of functional neuroanatomy is to develop an understanding of the neural circuitry underlying behavior. By knowing regional anatomy together with the functions of particular brain structures, the clinician can determine the location of nervous system damage in a patient who has a particular neurological or psychiatric impairment. Combined knowledge of what structures do and where they are located is essential for a complete understanding of nervous system organization. The term neuroanatomy is therefore misleading because it implies that knowledge of structure is sufficient to master this discipline. Indeed, in the study of neuroanatomy, structure and function are tightly interwoven, so much so that they should not be separated. The interrelationships between structure and function underlie functional localization, a key principle of nervous system organization.
This chapter examines the organization of the nervous system and the means to study it by developing the vocabulary to describe its regional anatomy. First, the cellular constituents of the nervous system are described briefly. Then the chapter focuses on the major regions of the nervous system and the functions of these regions. This background gives the reader insight into functional localization.
The nerve cell, or neuron, is the functional cellular unit of the nervous system. Neuroscientists strive to understand the myriad functions of the nervous system partly in terms of the interconnections between neurons. The other major cellular constituent of the nervous system is the neuroglial cell, or glia. Glia provide structural and metabolic support for neurons during development and in maturity.
It is estimated that there are about 100 billion neurons in the adult human brain. Although neurons come in different shapes and sizes, each has four morphologically specialized regions with particular functions: dendrites, cell body, axon, and axon terminals (Figure 1–2A). Dendrites receive information from other neurons. The cell body contains the nucleus and cellular organelles critical for the neuron’s survival and function. The cell body also receives information from other neurons and serves important integrative functions. The axon conducts information, which is encoded in the form of action potentials, to the axon terminal. Connections between two neurons in a neural circuit are made by the axon terminals of one and the dendrites and cell body of the other, at the synapse (discussed below).
FIGURE 1–2
Neurons are the functional cellular unit of the nervous system. A. A schematic nerve cell is shown, illustrating the dendrites, cell body, and axon. Dendritic spines are located on the dendrites. These are sites of excitatory synapses. Inhibitory synapses are located on the shaft of the dendrites, the cell body, and the initial segment. The axon can be seen to emerge from the cell body. The presynaptic terminals of the neuron are shown synapsing on the cell bodies of the postsynaptic neurons. The inset shows the spatial relations of three components of the synapse: the presynaptic axon terminal, the synaptic cleft, and the postsynaptic neuron. B. Selected examples of three neuron classes: (B1) unipolar, (B2) bipolar, and (B3) multipolar. (A, Adapted from Kandel ER, Schwartz JH, and Jessell TM, eds. Principles of Neural Science, 4th ed. New York, NY: McGraw-Hill, 2000. B, Reproduced with permission from from Cajal SR. Histologie du système nerveux de l’homme et des vertébres. 2 vols. Maloine, 1909-1911.)

Despite a wide range of morphology, we can distinguish three classes of neuron based on the configuration of their dendrites and axons: unipolar, bipolar, and multipolar (Figure 1–2B). These neurons were drawn by the distinguished Spanish neuroanatomist Santiago Ramón y Cajal at the beginning of the twentieth century. Unipolar neurons are the simplest in shape (Figure 1–2B1). They have no dendrites; the cell body of unipolar neurons receives and integrates incoming information. A single axon, which originates from the cell body, gives rise to multiple processes at the terminal. In the human nervous system, unipolar neurons are the least common. They control exocrine gland secretions and smooth muscle contractility.
Bipolar neurons have two processes that arise from opposite poles of the cell body (Figure 1–2B2). The flow of information in bipolar neurons is from one of the processes, which function like a dendrite, across the cell body to the other process, which functions like an axon. A bipolar neuron subtype is a pseudounipolar neuron (see Figure 6–2 top). During development the two processes of the embryonic bipolar neuron fuse into a single process in the pseudounipolar neuron, which bifurcates a short distance from the cell body. Many sensory neurons, such as those that transmit information about odors or touch to the brain, are bipolar and pseudounipolar neurons.
Multipolar neurons feature a complex array of dendrites on the cell body and a single axon that branches extensively (Figure 1–2B3). Most of the neurons in the brain and spinal cord are multipolar. Multipolar neurons that have long axons, with axon terminals located in distant sites, are termed projection neurons. Projection neurons mediate communication between regions of the nervous system and between the nervous system and peripheral targets, such as striated muscle cells. The neuron in Figure 1–2B3 is a particularly complex projection neuron. The terminals of this neuron are not shown because they are located far from the cell body. For this type of neuron in the human, the axon may be up to 1-m long, about 50,000 times the width of the cell body! Other multipolar neurons, commonly called interneurons, have short axons that remain in the same region of the nervous system in which the cell body is located. Interneurons help to process neuronal information within a local brain region.
Information flow along a neuron is polarized. The dendrites and cell body receive and integrate incoming information, which is transmitted along the axon to the terminals. Communication of information from one neuron to another also is polarized and occurs at specialized sites of contact called synapses. The neuron that sends information is the presynaptic neuron and the one that receives the information is the postsynaptic neuron. The information carried by the presynaptic neuron is most typically transduced at the synapse into a chemical signal that is received by specialized membrane receptors on the dendrites and cell body of the postsynaptic neuron.
The synapse consists of three distinct elements: (1) the presynaptic terminal, the axon terminal of the presynaptic neuron, (2) the synaptic cleft, the narrow intercellular space between the neurons, and (3) the receptive membrane of the postsynaptic neuron. Synapses are present on dendrites, the cell body, the initial segment of the axon, or the portion of the axon closest to the cell body, and the presynaptic axon terminal. Synapses located on different sites can serve different functions.
To send a message to its postsynaptic neurons, a presynaptic neuron releases neurotransmitter, packaged into vesicles, into the synaptic cleft. Neurotransmitters are small molecular weight compounds; among these are amino acids (eg, glutamate; glycine; and γ-aminobutyric acid, or GABA), acetylcholine, and monoaminergic compounds such as norepinephrine and serotonin. Larger molecules, such as peptides (eg, enkephalin and substance P), also can function as neurotransmitters. After release into the synaptic cleft, the neurotransmitter molecules diffuse across the cleft and bind to receptors on the postsynaptic membrane. Neurotransmitters change the permeability of particular ions across the neuronal membrane. A neurotransmitter can either excite the postsynaptic neuron by depolarizing it, or inhibit the neuron by hyperpolarizing it. For example, excitation can be produced by a neurotransmitter that increases the flow of sodium ions into a neuron (ie, depolarization), and inhibition can be produced by a neurotransmitter that increases the flow of chloride ions into a neuron (ie, hyperpolarization). Glutamate and acetylcholine typically excite neurons, whereas GABA and glycine typically inhibit neurons. Many neurotransmitters, like dopamine and serotonin, have more varied actions, exciting some neurons and inhibiting others. Their action depends on a myriad of factors, such as the particular receptor subtype that the neurotransmitter engages and whether the binding of the neurotransmitter leads directly to the change in ion permeability or if the change is mediated by the actions on second messengers and other intracellular signaling pathways (eg, G protein coupled receptors). For example, the dopamine receptor subtype 1 is depolarizing, whereas the type 2 receptor is hyperpolarizing; both act through G protein coupled mechanisms. A neurotransmitter can even have opposing actions on the same neuron depending on the composition of receptor subtypes on the neuron’s membrane. Action through second messengers and other intracellular signaling pathways can have short-term effects, such as changing membrane ion permeability, or long-term effects, such as gene expression. Many small molecules that produce strong effects on neurons are not packaged into vesicles; they are thought to act through diffusion. These compounds, for example, nitric oxide, are produced in the postsynaptic neuron and are thought to act as retrograde messengers that serve important regulatory functions on pre- and postsynaptic neurons, including maintaining and modulating the strength of synaptic connections. These actions are important for learning and memory.
Although chemical synaptic transmission is the most common way of sending messages from one neuron to another, purely electrical communication can occur between neurons. At such electrical synapses, there is direct cytoplasmic continuity between the presynaptic and postsynaptic neurons.
Glial cells comprise the other major cellular constituent of the nervous system; they outnumber neurons by about 10 to 1. Given this high ratio, the structural and metabolic support that glial cells provide for neurons must be a formidable task! There are two major classes of glia: microglia and macroglia. Microglia subserve a phagocytic or scavenger role, responding to nervous system infection or damage. They are rapidly mobilized—they become activated—in response to different pathophysiological conditions and trauma. Activated microglia can destroy invading microorganisms, remove debris, and promote tissue repair. Interestingly, they also mediate changes in neuronal properties after nervous system damage; sometimes maladaptive changes, so they may also hinder recovery after injury. For example, neurons often become hyperexcitable after nervous system damage, and microglia can be involved in this process. Macroglia, of which there are four separate types—oligodendrocytes, Schwann cells, astrocytes, and ependymal cells—have a variety of support and nutritive functions. Schwann cells and oligodendrocytes form the myelin sheath around peripheral and central axons, respectively (Figures 1–2A and 1–3). The myelin sheath increases the velocity of action potential conduction. It is whitish in appearance because it is rich in a fatty substance called myelin, which is composed of many different kinds of myelin proteins. Schwann cells also play important roles in organizing the formation of the connective tissue sheaths surrounding peripheral nerves during development and in axon regeneration following damage in maturity. Astrocytes have important structural and metabolic functions. For example, in the developing nervous system, astrocytes act as scaffolds for growing axons and guides for migrating immature neurons. Many synapses are associated with astrocyte processes that may monitor synaptic actions and provide chemical feedback. Astrocytes also contribute to the blood-brain barrier, which protects the vulnerable environment of the brain from invasion of chemical from the periphery, which can influence neuronal firing. The last class of macroglia, ependymal cells, line fluid-filled cavities in the central nervous system (see below). They play an important role in regulating the flow of chemicals from these cavities into the brain.
FIGURE 1–3
Astrocytes and oligodendrocytes are the most ubiquitous types of glial cells in the central nervous system. Parts A and B are histological sections showing examples of these cell types. A. An astrocyte (green) is shown enveloping a neuronal cell body (red). B. Oligodendrocytes are shown forming the myelin sheaths surrounding axons. A blue stain (DAPI) marks nuclei in the cell bodies. The processes (green) are stained for an important component of the myelin sheath, myelin basic protein (MBP). (Part A, Image courtesy of Ellisman M and Bushong E, Univ. California, San Diego. Allen NJ, Barres BA. Neuroscience Glia: More than just brain glue. Nature. 2009;457 [7230]:675-677. Part B, Reproduced with permission from Lee PR, Fields RD. Regulation of myelin genes implicated in psychiatric disorders by functional activity in axons. Front Neuroanat. 2009;3:4. Part C, Adapted from Kandel ER, Schwartz JS, and Jessell TM, eds. Principles of Neural Science, 4th ed. New York, NY: McGraw-Hill, 2000.).

Neurons and glial cells of the nervous system are organized into two anatomically separate but functionally interdependent parts: the peripheral and the central nervous systems (Figure 1–4A). The peripheral nervous system is subdivided into somatic and autonomic divisions. The somatic division contains the sensory neurons that innervate the skin, muscles, and joints. These neurons detect and, in turn, inform the central nervous system of stimuli. This division also contains the axons of motor neurons that innervate skeletal muscle, although the cell bodies of motor neurons lie within the central nervous system. These axons transmit control signals to muscle to regulate the force of muscle contraction. The autonomic division contains the neurons that innervate glands and the smooth muscle of the viscera and blood vessels (see Chapter 15). This division, with its separate sympathetic, parasympathetic, and enteric subdivisions, regulates body functions based, in part, on information about the body’s internal state.
FIGURE 1–4
A. Location of the central and peripheral nervous system in the body. Major peripheral nerves are shown in yellow. B. The brain and spinal cord, viewed laterally. C. There are seven major divisions of the central nervous system: (1) cerebral hemispheres, (2) diencephalon, (3) midbrain, (4) pons, (5) cerebellum, (6) medulla, and (7) spinal cord. The midbrain, pons, and medulla comprise the brain stem.

The central nervous system consists of the spinal cord and brain (Figure 1–4B), and the brain is further subdivided into the medulla, pons, cerebellum, midbrain, diencephalon, and cerebral hemispheres (Figure 1–4C). Within each of the seven central nervous system divisions resides a component of the ventricular system, a labyrinth of fluid-filled cavities that serve various supportive functions (see Figure 1–13). Box 1–1 shows how all of the divisions of the central nervous system and the components of the ventricular system are present from very early in development, from about the first month after conception.
Box 1–1 Development of the Basic Plan of the Brain and Spinal Cord
The central nervous system develops from a specialized portion of the embryonic ectoderm, the neural plate. Originally a flattened sheet of cells, the neural plate forms a tube-like structure—termed the neural tube—as the neurons and glial cells proliferate. The walls of the neural tube form the neuronal structure of the central nervous system. The cavity in the neural tube forms the ventricular system.
Very early in development the rostral portion of the neural tube forms the three hollow swellings, or vesicles, corresponding to where there is an enormous proliferation of developing neurons (Figure 1–5): (1) the prosencephalon, or forebrain, (2) the mesencephalon, or midbrain, and (3) the rhombencephalon, or hindbrain. The caudal portion of the neural tube remains relatively undifferentiated and forms the spinal cord. Two secondary vesicles emerge from the prosencephalon later in development, the telencephalon (or cerebral hemisphere) and the diencephalon (or thalamus and hypothalamus). Whereas the mesencephalon remains undivided throughout further brain development, the rhombencephalon gives rise to the metencephalon (or pons and cerebellum) and the myelencephalon (or medulla). The five brain vesicles and primitive spinal cord, already identifiable by the fifth week of fetal life, give rise to the seven major divisions of the central nervous system (see Figure 1–4).
The complex configuration of the mature brain is determined in part by how the developing brain bends, or flexes. Flexures occur because proliferation of cells in the brain stem and cerebral hemispheres is enormous, and the space that the developing brain occupies in the cranium is constrained. At the three-vesicle stage, there are two prominent flexures: the cervical flexure, at the junction of the spinal cord and the caudal hindbrain (or future medulla), and the cephalic flexure, at the level of the midbrain (Figure 1–5, bottom). At the five-vesicle stage, a third flexure becomes prominent, the pontine flexure. By birth the cervical and pontine flexures have straightened out. The cephalic flexure, however, remains prominent and causes the longitudinal axis of the forebrain to deviate from that of the midbrain, hindbrain, and spinal cord (see Figure 1–16B).
The large cavities within the cerebral vesicles develop into the ventricular system of the brain, and the caudal cavity becomes the central canal of the spinal cord (Figure 1–5). The ventricular system contains cerebrospinal fluid, which is produced mainly by the choroid plexus (see Chapter 3). As the brain vesicles develop, the cavity within the cerebral hemispheres divides into the two lateral ventricles (formerly termed the first and second ventricles) and the third ventricle (Figure 1–5B). The lateral ventricles, which develop as outpouchings from the rostral portion of the third ventricle, are each interconnected with the third ventricle by an interventricular foramen (of Monro) (Figure 1–5, inset). The fourth ventricle, the most caudal ventricle, develops from the cavity within the hindbrain. It is connected to the third ventricle by the cerebral aqueduct (of Sylvius) and merges caudally with the central canal (of the caudal medulla and spinal cord).
Cerebrospinal fluid normally exits from the ventricular system into the space overlying the central nervous system’s surface through foramina in the fourth ventricle (discussed in Chapter 3). (The central canal does not have such an aperture for the outflow of cerebrospinal fluid.) Pathological processes can prevent flow of cerebrospinal fluid from the ventricular system. For example, later in development the cerebral aqueduct becomes narrowed because of cell proliferation in the midbrain. Its narrow diameter makes it vulnerable to the constricting effects of congenital abnormalities, tumors, or swelling from trauma. Occlusion can occur; however, cerebrospinal fluid continues to be produced despite occlusion. If occlusion occurs before the bones of the skull are fused (ie, in embryonic life or in infancy), ventricular volume will increase, the brain will enlarge rostral to the occlusion, and head size will increase. This condition is called hydrocephalus. If occlusion occurs after the bones of the skull are fused, ventricular size cannot increase without increasing intracranial pressure. This is a life-threatening condition.
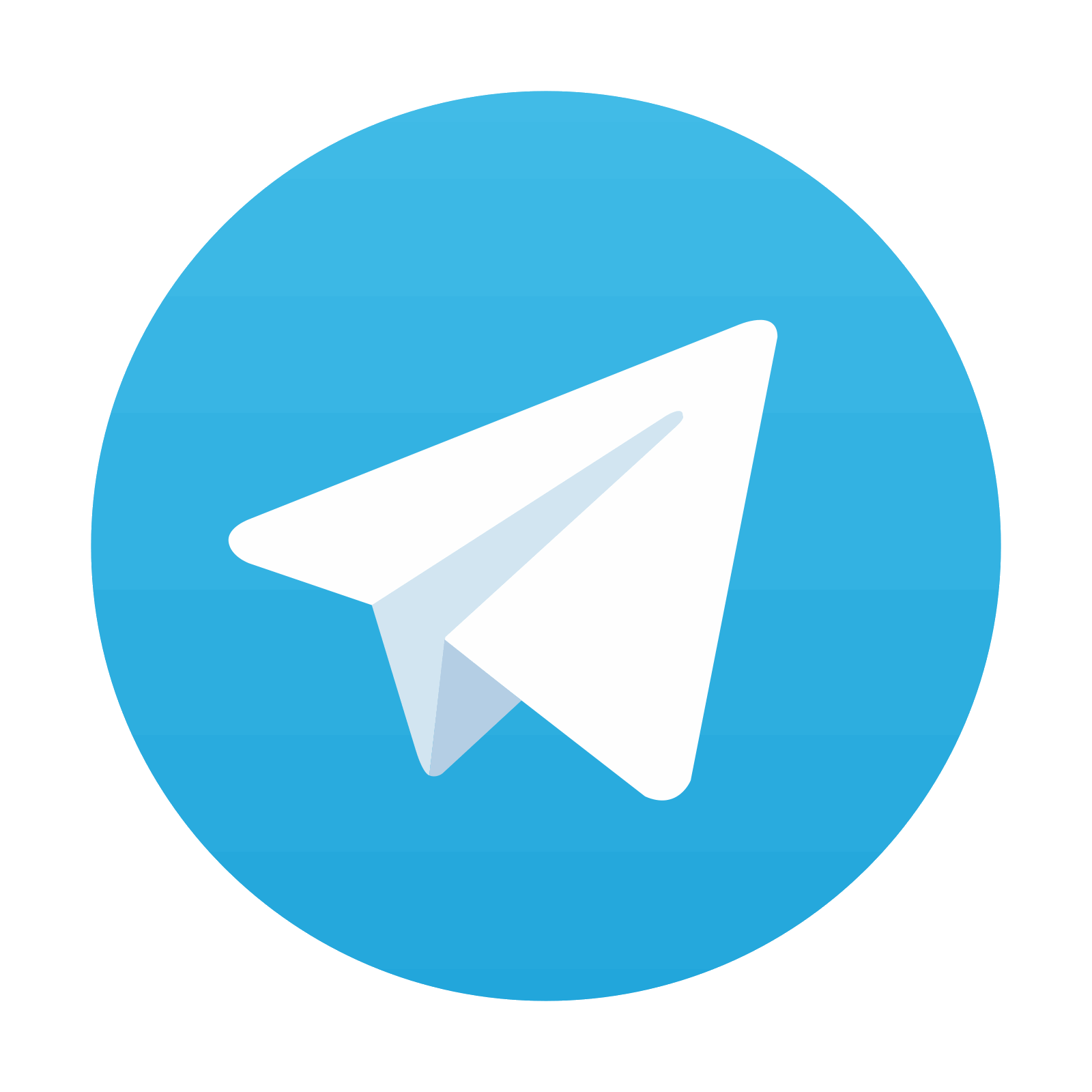
Stay updated, free articles. Join our Telegram channel
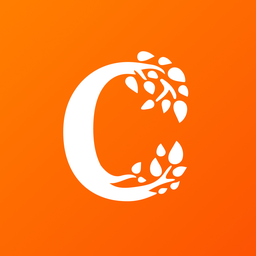
Full access? Get Clinical Tree
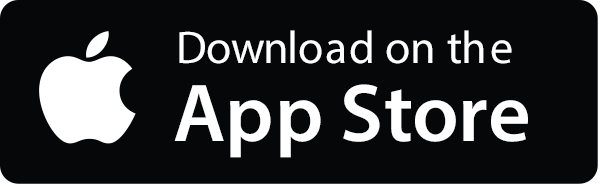
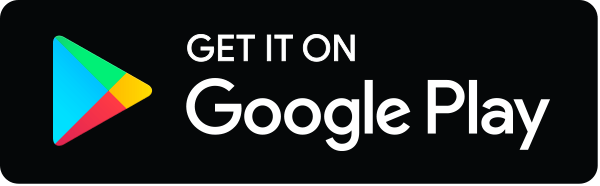