Derangement
EEG findings
Benzodiazepine or barbiturate use
Diffuse beta activity
Hepatic failure
Early—slowed alpha rhythm
Late—persistent theta or delta activity
Triphasic wave pattern—2–4 Hz bilaterally synchronous waves with a triphasic morphology
Renal failure
Early—slowed alpha rhythm
Late—nearly persistent theta or delta activity
Triphasic waves—also present
Hyponatremia
Early—slowposterior alpha rhythm
Late—generalized slow activity
High-voltage IRDA
Epileptiform discharges are uncommon even with seizures
Hypernatremia
Theta activity
Often no EEG changes at all
Hypoglycemia
Enhanced response to hyperventilation with FIRDA longer after cessation of hyperventilation
Glucose 50–80 mg/dl: slowing of alpha rhythm
Glucose below 40 mg/dl diffuse theta and delta with IRDA activity
Epileptiform discharges are uncommon even with seizures
Hyperglycemia
Changes typically not seen until glucose greater than 400 mg/dl
Sporadic epileptiform discharges
Hypothermia
Core temperature 29–30 °C—diffuse theta and delta
Core temperature 20–22 °C—burst suppression pattern
Core temperature <18 °C—electrocerebral silence
Paroxysmal and Ictal EEG Patterns
According to the International Federation of Societies for Electroencephalography and Clinical Neurophysiology (IFSECN) , a transient is an event that clearly stands out against the background EEG and can be a single wave or a sequence of two or more waves. Spikes are transients which are considered epileptiform and are clearly distinguished from the background with a pointed peak and duration between 20 and 70 ms and a sharp wave is a transient clearly distinguished from the background with a pointed peak and duration between 70 and 200 ms [14]. Spikes and sharp waves are closely related phenomenon, both of which are associated with an epileptic seizure disorder, although both transients may occur with no prior history of epileptic seizure disorder [1]. These epileptiform and highly epileptogenic potentials are seen in patients with and without structural brain lesions. These discharges are often seen interictally in patients with brain tumors and epilepsy. They are often localized to the region of the brain tumor but in multifocal structural lesions such as brain metastasis, multifocal epileptiform discharges can be seen. It is uncommon for this epileptiform activity to be the only EEG abnormality associated with a tumor. Typically, some degree of focal slowing is present when epileptiform activity is present [13, 15].
While epileptiform activity is commonly seen with indolent or static lesions, periodic lateralized epileptiform discharges are more commonly seen with an acute lesion [13]. Periodic lateralized epileptiform discharges (PLEDs) are sharp waves, spike waves, or more complex wave forms recurring periodically every 1–2 s with a return to background between discharges and occupying a relatively large area of the hemicranium [13, 16]. They can be due to a variety of structural lesions including neoplasms, hematomas, and infections. While stroke is the most common etiology of PLEDs, 12% of patients with PLEDs have brain tumors, almost all of which are supratentorial brain tumors. PLEDs are considered to be on the ictal-interictal spectrum and are highly associated with conversion to frank seizure activity. Almost 50% of patients with brain tumors and PLEDs have seizures [16].
When a patient has a seizure that persists for a sufficient length of time or repeats frequently enough that recovery between attacks does not occur, it is termed status epilepticus. Mortality with status epilepticus is up to 20%. Convulsive status epilepticus accounts for nearly half of all status epilepticus [17]. Over 4% of patients who present with status epilepticus have brain tumors. In patients who present with status epilepticus with no preexisting history of seizure, almost 9% have brain tumors [18]. Patients with structural brain lesions more often have refractory status epilepticus and over 5% of patients presenting with refractory status epilepticus have this condition secondary to a brain tumor [19].
At times, status epilepticus is partial (as opposed to generalized) in nature. Epilepsia partialis continua is a partial somatomotor status epilepticus that is often seen in the setting of brain tumors. Between 11 and 15% of patients who present with epilepsia partialis continua have brain tumors as the etiology. EEG findings with this can be variable depending on the area of focus and, at times, the EEG can be normal given that it takes a sufficient amount of cortex to elicit a response on EEG [20, 21].
A common cause of altered mental status in the brain tumor population is non-convulsive status epilepticus (NCSE) . NCSE can be defined as status epilepticus with reduced or altered consciousness, behavioral and vegetative abnormalities, or subjective symptoms with no major convulsive movements [22]. NCSE is the cause of altered mental status in over 5% of tumor patients [23]. NCSE is more common in patients with gliomas than in those with metastatic tumors [24]. Even in patients with systemic cancer but no brain metastasis, NCSE was the cause of altered mental status in 6% of patients [23]. EEG monitoring can be particularly helpful in the diagnosis of seizures in these patients and should be utilized in any cancer patient with altered mental status [25].
EEG Changes by Tumor Type
EEG changes in patients with brain tumors depend on several factors, including location, size, and growth rate. Although EEG patterns are not specific for the histologic pathology of the tumor, some general correlations can be made.
Extra-axial tumors that are slow growing, like meningiomas, are less likely to produce EEG abnormalities. Meningiomas located in the convexities, especially parasagittally, are more likely to cause changes like focal theta, FIRDA, and diminished or altered frontal beta rhythms. Rolandic meningiomas cause epileptiform discharges in up to 45% of patients with similar incidence of seizure. Meningiomas of other regions are less likely to cause EEG effects; however, approximately 25% of patients with meningiomas present with seizures [26, 27].
Indolent gliomas are more likely to cause EEG changes. Glioneural tumors including gangliogliomas and dysembryoplastic neuroepithelial tumors (DNETs) have the highest rates of epilepsy with 90–100% of patients having seizures, often pharmacoresistant [8, 28]. Diffuse low-grade gliomas cause seizures in 60–88% of patients [8, 29]. Focal polymorphic slow activity is usually a later finding and can occur intermittently. This slowing becomes increasingly persistent as the tumor progresses [30].
Rapidly growing parenchymal tumors , such as glioblastomas, are more likely to cause marked abnormalities on EEG but are less likely to cause seizures. Glioblastomas and other rapidly growing and destructive tumors cause prominent and focal polymorphic delta activity with marked alterations of background rhythms unilaterally and often bilaterally. Areas of necrosis can be electrically silent causing regions of attenuation. FIRDA occurs more frequently in glioblastoma than in other types of supratentorial tumors. With glioblastoma, epileptiform discharges are not present as frequently as they are with more indolent gliomas but PLEDS are more likely to occur [13]. Seizure rates in glioblastoma are approximately 30–50%, which is significantly lower than what is seen with lower grade gliomas [8, 31, 32].
Metastatic tumors to brain occur most commonly with cancers of the lung, breast, kidney, and melanoma. The EEG findings tend to be lateralized to the side of the metastasis. Larger or multiple metastatic lesions cause more EEG abnormalities than do small solitary lesions. The slow activity in metastatic lesions tends to be more theta range, compared to delta slow activity present with glioblastomas. EEG slow activity in metastatic disease also tends to be more episodic rather than persistent as seen with glioblastomas [33]. Metastatic lesions cause epileptogenic discharges in about one-third of patients and seizures in nearly one-half. Overall, metastatic lesions tend to cause more benign EEG changes than do gliomas of any grade [34].
EEG Changes by Tumor Location
EEG findings can vary by location of the tumor. Since the EEG reflects cortical neuron activity, hemispheric tumors affect EEG more markedly and reliably than do deeper tumors and tumors of the posterior fossa.
Frontal lobe tumors often cause local high-voltage delta slow activity. The slow activity can be continuous or episodic and bifrontal with a FIRDA pattern. FIRDA is more common with frontal tumors than in tumors of other locations. The background activity can be relatively preserved in frontal tumors. Epileptiform discharges are seen in almost one-half of frontal tumor patients [26].
Temporal tumors are the easiest to localize with EEG and display moderate to high-voltage focal polymorphic delta slowing lateralized over the tumor in over 80% of cases. In a majority of cases the slow activity is localized to the region of the tumor [33]. The slow activity recorded with temporal tumors tends to be continuous rather than intermittent. Temporal lobe tumors are also likely to cause FIRDA, but less so than frontal lobe tumors [26]. Background rhythms are more likely to be altered in posterior temporal lesions than in anterior temporal lesions.
Parietal tumors are less likely to cause EEG changes than are other supratentorial tumors. Slow activity can be local or more widespread and is more likely to be theta range, only of a moderate voltage, and intermittent or continuous. The background rhythms tend to be disturbed with parietal tumors [33].
Occipital tumors tend to cause focal polymorphic delta activity. Slow activity in surrounding temporal and parietal regions is often seen in conjunction with this. The posterior alpha rhythm is frequently absent ipsilaterally [1].
Tumors of subcortical structures vary in their findings and can be fairly non-localizing. Tumors of deep structures including the hypothalamus, fornix, basal ganglia, internal capsule, and corpus callosum characteristically cause intermittent slow activity. The slow activity is often monorhythmic and bilateral in an IRDA pattern [35]. Up to one-fourth of patients with lesions in these regions will have normal EEG findings [13]. Tumors of the sellar region typically do not cause EEG findings until they expand and obstruct the third ventricle, only then causing IRDA [36]. Thalamic tumors can cause IRDA but have also been shown to cause attenuation, disorganization of background frequencies, and slow activity of the ipsilateral alpha rhythm [37].
Tumors of the third ventricle and posterior fossa tend to be variable and are often normal on EEG. Abnormalities are more frequently found with obstruction of cerebrospinal fluid flow causing obstructive hydrocephalus. If an abnormal EEG is seen, the most common pattern is that of IRDA [1].
EEG Changes with Tumor Treatment
Iatrogenic changes are also seen on EEG in response to treatment therapies. Some are well characterized and others less so. EEG is useful in diagnosing epileptogenic potential with new cancer treatments and chemotherapeutic agents.
Brain irradiation occurs in two forms, whole brain radiation (WBRT) and partial brain radiation . WBRT can cause an acute or delayed reaction with cognitive decline and neurologic deficits. It can also cause generalized slowing of the background rhythms, IRDA, or even focal polymorphic delta slowing due to necrosis. Partial brain radiation can cause necrosis lending to focal slow activity, epileptogenic discharges, and even seizures [38–40].
Numerous medications for cancer treatment have an associated incidence of central nervous system toxicity. Some drugs cause diffuse dysfunction and slow activity on EEG while others are frankly epileptogenic. Table 3.2 lists known electrographic changes associated with common cancer treatments [41, 42].
Table 3.2
Cancer treatments and their associated EEG findings
Cancer treatment | EEG findings |
---|---|
Busulfan | Diffuse and focal delta |
Resolves within days of treatment discontinuation | |
Seizures common and require prophylactic antiepileptic drug administration | |
Chimeric antigen receptor T-cell therapy | Slow background activity |
Epileptogenic discharges and increased seizure frequency | |
Cytarabine | Diffuse theta and delta activity |
Ifosfamide | Slow alpha rhythm with increased theta and delta |
Triphasic waves | |
Epileptogenic discharges and increased seizure frequency | |
EEG and encephalopathy improve with benzodiazepine administration | |
Interferons | Diffuse theta and delta activity |
FIRDA activity | |
Methotrexate | Both diffuse and localized delta activity |
Seizure incidence increased | |
Pacitaxel | Diffuse theta activity |
Vincristine | Diffuse theta and delta activity |
Epileptogenic discharges | |
Increased seizure frequency with focal seizures |
EEG Changes with Autoimmune and Paraneoplastic Disorders
Paraneoplastic limbic encephalitis is becoming increasingly recognized with the commercial availability of antibody assays. Any paraneoplastic encephalitis involving the cortex or limbic system can cause EEG abnormalities and seizures. There are several autoantibody syndromes that are commonly associated with paraneoplastic epilepsy. These cause marked EEG abnormalities that are sometimes reversible with treatment of the underlying malignancy. Up to 100% of patients with paraneoplastic limbic encephalitis have an abnormal EEG, with seizures occurring in over 50% of these patients. EEG abnormalities include diffuse slowing, focal slowing, multifocal slowing, focal or multifocal epileptiform discharges, periodic lateralized epileptiform discharges (PLEDs) , and seizures [43, 44]. Table 3.3 lists autoantibodies and their associated EEG findings [45–50].
Table 3.3
Autoantibodies and their associated EEG findings
Antibody | Associated cancers | EEG findings |
---|---|---|
Anti-Hu | Small cell lung cancer | 10% EEGs abnormal Temporal, bitemporal, or multifocal epileptiform discharges Seizures, epilepsia partialis continua, status epilepticus |
Anti-Ma | Testicular germ cell tumor Breast cancer Non-small cell lung cancer | Epileptiform discharges Seizures and status epilepticus |
NMDA | Ovarian teratoma | Irregular or rhythmic delta activity Extreme delta brush pattern Up to 70% with seizures or status epilepticus Tonic, focal, or generalized seizures |
AMPA | Thymus Breast Lung | Temporal or bitemporal epileptiform discharges or seizures Diffuse, focal, or multifocal slow activity |
GABA-B | Small cell lung cancer | Diffuse slow activity PLEDs Up to 100% with seizures |
VGKC | Small cell lung cancer Often no tumor found | Temporal or bitemporal epileptiform discharges Faciobrachial dystonic seizures, tonic seizures, temporal seizures Diffuse slow activity |
Electromyography
History
The groundwork set by Alessandro Volta and Luigi Galvani paved the way for later visionaries in the field of peripheral electrodiagnosis [51, 52]. In 1833, Guillaume-Benjamin Duchenne used cloth-covered electrodes to stimulate nerves from the surface of the skin [53, 54]. Later, in 1850, Helmholtz successfully measured the conduction velocity of a nerve impulse of a frog, and later a human median nerve [54, 55]. In 1882, Wilhelm Erb devised a formula for polar contraction in normal and abnormal nerve states, and is generally cited as the founder of classic electrodiagnostics [54, 56]. On the electromyography front, a sensitive recording apparatus was needed for progress in the study of muscle action potentials. In 1922, Herbert Spencer Gasser and Joseph Erlanger overcame the limitations of a galvanometer with the advent of a cathode ray oscilloscope and laid the ground of the modern electrodiagnostic era [54, 57]. The first electromyogram (EMG) in a patient was performed by R. Proebster in 1928 and, following this, the field continued to expand into common clinical use [54, 58].
The demand for electrical testing of peripheral nerves grew with the two World Wars as physicians treating war casualties needed to know the extent of damaged nerves and the status of regeneration. This sparked a greater interest in the field and the need for peripheral diagnostics was realized. The First International Congress of Electromyography was held in 1961 in Pavia, Italy, and helped standardize values and the understanding of current peripheral electrodiagnostic studies [54]. Today, we continue to use electromyography and nerve conduction measurements to aid in the diagnosis of peripheral nervous system disorders.
Technical Component
EMG is a method of recording motor unit potentials with extracellular electrodes. This study is typically performed after a nerve conduction study (NCS) has narrowed the clinical diagnosis and suggested muscles that may be pathologically involved. To perform an EMG, a needle electrode is inserted into the muscle of interest. See Fig. 3.1. The needle may have a reference electrode embedded within it (concentric needle) or a monopolar needle with a surface skin reference electrode can be used. The tip of the needle is the active recording electrode and a triphasic waveform is produced as the muscle action potential approaches, reaches, and leaves the recording electrode. The needle is connected to an EMG machine which records and amplifies the signal and provides a visual and auditory representation of the signal [54, 59].


Fig. 3.1
Electromyography showing a needle insertion with EMG tracing
To perform the test, a needle electrode is placed in the muscle and electrical activity is noted during the insertion. Insertional activity from initial needle insertion should be only a few hundred milliseconds in duration. Insertional activity can be classified as normal, decreased (seen in fibrotic muscle disease) or increased (seen in denervated muscle). Next, the muscle is evaluated while it is at rest for any spontaneous activity. All muscle fibers of a given motor unit will fire at the same time as action potentials are an all-or-none response. Damaged and denervated muscle fibers become unstable and no longer fire with the rest of their motor unit. These damaged fibers can fire spontaneously with no external stimuli, producing spontaneous activity. Fibrillation potentials and positive sharp waves are the most common findings when assessing for spontaneous activity, and typically signify active denervation [54, 59, 60]. The patient is then asked to perform mild voluntary contraction of the muscle to evaluate motor unit potentials. Motor unit action potentials (MUAP) have typical morphology and characteristics. The duration reflects the number of muscle fibers within the motor unit and is typically 5–15 ms from initial take-off to the return to baseline. The number of phases, or times the waveform crosses baseline, is typically 2–4 in normal MUAPs. Polyphasia can be seen in both neuropathic and myopathic disorders. The amplitude of a MUAP is typically 100 μV–2 mV, and reflects only the few fibers that are nearest to the needle. After the MUAP is assessed with mild voluntary contraction, the patient is asked to perform maximal voluntary contraction of the muscle in order to evaluate recruitment and interference. Normally with muscle contracture, motor units can increase muscle force by increasing the firing rate or by recruiting additional motor units to fire. Decreased recruitment occurs when there is a loss of MUAPs, typically through axonal loss or conduction block, where additional units cannot be recruited easily. In contrast, increased or early recruitment typically occurs with myopathic processes where the individual muscle fibers within a unit cannot produce enough force and require additional motor units to generate a small amount of force. Once all of these factors are evaluated, the needle is moved to another location to repeat the above maneuver. The needle electrode samples muscle motor unit potentials in close proximity to the location of needle insertion; thus, multiple sites must be examined to have a thorough sampling of an area of interest. Typically, sampling is done in at least four directions from any given puncture site and multiple puncture sites are selected in one or several muscles of interest [54, 59, 60].
NCS can be performed to evaluate both nerve compound motor action potentials (CMAP) and sensory nerve action potentials (SNAP) . CMAPs are measured in millivolts (mV) and are very large compared to SNAPs which are measured in microvolts (µV). While these have some differences in interpretation, much of the technical components remain the same [54, 59].
A stimulating probe is typically used with fixed surface electrodes that consist of a cathode (negative pole) and anode (positive pole) that are two to three centimeters apart. The cathode is positioned closer to the recording site. An electrical current is introduced into the stimulating probe. The current flows from the anode to the cathode, collecting negative charges between the cathode and the underlying nerve surface and depolarizing the underlying nerve. To record the stimulus for a motor nerve, an active recording surface electrode is placed over the belly of a muscle innervated by the nerve of interest, and a second inactive recording surface electrode is placed over the tendon of the muscle. When a stimulating current is given from the stimulating probe, the nerve is depolarized and the propagating muscle action potential is recorded at active recording electrode which is at the motor end point. See Fig. 3.2. Sensory nerves can be stimulated orthodromically (distal-to-proximal or in the direction of sensory flow) or antidromically (proximal-to-distal or opposite of the direction of sensory flow). Orthodromic recording is more commonly used; the active recording surface electrode is placed over the nerve and the second, inactive, recording surface electrode is placed at a remote site. Again, the stimulating probe depolarizes the nerve and propagates an action potential recorded at the given location on the sensory nerve [54, 59].


Fig. 3.2
Nerve conduction velocity . Depicting the stimulator with recording electrodes on the thenar eminence
Several parameters are recorded and analyzed in a NCS. Amplitude (measured from baseline to the negative peak) reflects the number of muscle fibers that are depolarized. Duration (measured from the initial deflection from baseline to the first crossing of baseline) is a measure of synchrony of the muscle fibers. Conduction velocity (the distance traveled divided by the nerve conduction time) measures the speed of the fastest conducting axons. Latency (onset latency the time from the stimulus to the initial deflection from baseline), or peak latency (the time from the stimulus to the midpoint of the first negative peak) reflects the time from stimulation until a response is recorded at recording electrode. With motor studies, the distal motor latency includes the conduction time along the distal nerve, the neuromuscular junction (NMJ) transmission time, and the muscle depolarization time, necessitating two stimulation sites in order to subtract the time across the NMJ and muscle. With sensory studies, only one stimulation is needed to measure the latency and velocity [60].
There are several basic abnormal nerve conduction patterns. With neuropathic lesions one can see axonal loss, with reduced amplitude being the primary finding, or demyelination, with slowed velocity and latency. In myopathic disorders, the sensory NCS remain normal, as do the distal latencies and conduction velocities, with an occasional decrease in CMAP amplitude. These patterns are important in understanding etiologies of disease and correlating this with the clinical picture. See Table 3.4.
Table 3.4
Comparison of neuropathy versus myopathy
NCV | CMAP | SNAP | MUAP | |
---|---|---|---|---|
Neuropathy—axonal | Normal | Decreased | Decreased | Large |
Neuropathy—demyelinating | Decreased | Normal | Normal | Normal |
Myopathy | Normal | Decreased | Normal | Small |
Indications and Contraindications
NCS and EMG are used to diagnose disorders of the peripheral nervous system (PNS), including disorders of primary motor neurons (anterior horn cells), sensory neurons (dorsal root ganglia), nerve roots, brachial and lumbosacral plexuses, peripheral nerves, neuromuscular junctions, and muscles. Patients with peripheral pain, paresthesias, sensory loss, atrophy, and weakness are routinely tested via EMG and NCS in order to better delineate an etiology and treatment plan. EMG and NCS have become a staple in our armamentarium and continue to provide useful diagnostic information in cancer patients.
At this time, there are no true contraindications of NCS. They are routinely performed in patients with pacemakers, cochlear implants, vagus nerve stimulators, deep brain stimulators, and other electric hardware as there are insufficient data to suggest significant risk to the patient [54, 59]. Although there is no clearly defined risk, a discussion with the patient’s cardiologist may be advised if the NCS is to be performed close to the chest wall, or if dysfunction of the pacemaker may be life threatening [61, 62].
Patients with a bleeding tendency should be screened prior to performing routine EMG examination. The study maybe contraindicated in patients with untreated hemophilia or severe thrombocytopenia (typically considered platelets <20,000/mm [3]). Patients with iatrogenic blood thinning due to oral or intravenous anticoagulation should not undergo EMG until there is some degree of reversal of the prothrombin time or partial thromboplastin time. In addition to bleeding tendency, EMG should not be performed in an area with local skin or soft tissue infection as there is a risk of bacteremia [54].
Local Cancer Invasion
Tumors can enter the peripheral nervous system by direct invasion or hematogenous spread. The tumor can then externally compress the nerve fibers or invade them. Neural compression is more common with many cancers causing local trauma to the nerve with resultant neurologic symptoms and deficits. Neural invasion is less common but has been demonstrated with prostate cancer, breast cancer, lung cancer, pancreatic cancer, and lymphoma [63].
Leptomeningeal metastasis or epidural tumors can cause radiculopathy with irritation or direct compression of the nerve roots. Plexopathies are quite frequent in approximately 1% of cancer patients with some metastatic involvement of their plexi [64]. Invasion of the lumbosacral plexus is more common and is often due to direct extension of abdominal and pelvic tumors including cervical cancer, colorectal cancer, bladder cancer, and retroperitoneal sarcomas. Invasion of the brachial plexus is typically due to breast and lung cancer. The lower trunk of the brachial plexus is most commonly involved due to its proximity to the lateral axillary lymph nodes [64]. Peripheral neuropathies are less frequent, but can be seen with peripheral nervous system infiltration from lymphoma called neurolymphomatosis. This is a well-known disorder and has been described with both B-cell and T-cell lymphomas [65]. It tends to invade multiple nerves or nerve roots and presents clinically as a polyneuropathy or polyradiculopathy [66]. Mononeuropathies are less common with local cancer invasion, but can occur with extension of bony metastasis. Malignant nerve sheath tumors that typically occur in the setting of neurofibromatosis I, or as a result of radiation therapy, can also cause a mononeuropathy [65].
The neurologic symptoms and deficits depend on the topography of the nerve involvement. Electrodiagnostic testing with EMG and NCS can be valuable in localizing nerve involvement to determine if the pathology is in the root, plexus, peripheral nerve, or neuromuscular junction. Although neurophysiologic testing does not distinguish local cancer invasion from other types of nerve damage, it can help localize the region of pathology so that a specific region can be imaged or biopsied for further investigation and prognostication.
Paraneoplastic and Antibody Mediated
Most paraneoplastic disorders begin acutely or subacutely and progress over time often with some stabilization late in the disease process. The current concept of paraneoplastic syndromes is that the primary tumor ectopically expresses an antigen that is identical in structure to a neural antigen but seen by the host immune system as foreign, thus eliciting an immune attack on both the antigen and the neural structure. The central nervous system or peripheral nervous system can be attacked, leaving the patient with debilitating symptoms. The incidence of paraneoplastic peripheral neuropathy is thought to be 6–8%, with most paraneoplastic neuropathies being sensorimotor and axonal [67]. Paraneoplastic disorders can also occur at the level of the neuromuscular junction or muscle. While antibodies and tissue pathology are more specific, EMG and NCS can be a valuable tool in the diagnosis of peripheral paraneoplastic disorders. Here we describe some common paraneoplastic and antibody-mediated disorders of the peripheral nervous system (motor neuron, nerve, NMJ and muscle) and their associated neurophysiologic findings. See Table 3.5.
Table 3.5
Autoantibody , paraneoplastic , and paraproteinemic diseases of the peripheral nervous system and associated EMG/NCV findings
Associated cancer | NCV | EMG | |
---|---|---|---|
AIDP | Hodgkin lymphoma, lung cancer, breast cancer | Prolonged F-wave | Normal or decreased recruitment |
Increased distal latencies | |||
Conduction block | |||
CIDP | Lymphoma, leukemia, melanoma, paraproteinemia | Absent or prolonged F-wave | |
Increased distal latencies | |||
Abnormal temporal dispersion, conduction block | |||
Paraproteinemic neuropathy | Amyloid, multiple myeloma, MGUS, Waldenström’s macroglobulinemia | Decreased motor and sensory CMAP | Spontaneous activity an acute denervation |
Anti-CV2 | Small cell lung cancer | Decrease motor sensory CMAP | |
Anti-Hu | Small cell lung cancer | Decreased sensory CMAP; Normal motor CMAP | Normal or active denervation |
Mononeuritis multiplex | Vasculitic neuropathy, small cell lung cancer, uterine cancer, lymphoma, prostate cancer | Decreased sensory CMAP | |
Neuromyotonia | Thymoma, lymphoma, small cell lung cancer | Spontaneous irregular firing of single or MUAP | |
Persist during sleep and general anesthesia | |||
Stiff person syndrome | Breast cancer, small cell lung cancer, Hodgkin lymphoma, colon cancer | Sustained continuous MUAP, disappeared during sleep and general anesthesia | |
Lambert-Eaton myasthenic syndrome | Small cell lung cancer | Decrement with slow rate repetitive nerve stimulation; 3–5 Hz | Single-fiber EMG-increased jitter |
High rate stimulation; 20–50 Hz results in increased CMAP | |||
Myasthenia gravis | Thymoma, lymphoma | Slow rate repetitive nerve stem; 3–5 Hz decrement in CMAP amplitude | Single-fiber EMG-increased jitter |
High rate stimulation-no increment |
Acute Inflammatory Demyelinating Polyneuropathy
An acute inflammatory demyelinating polyneuropathy (AIDP or Guillain–Barre syndrome ) with acute to subacute weakness and sensory features has an increased incidence in patients with Hodgkin lymphoma as well as various types of solid cancers [68, 69]. With AIDP, typical findings include prolonged or absent F waves followed by increased distal latencies and conduction block of motor responses. Significant slowing of the motor nerve conduction velocities is not seen until later in the disease course. Sensory NCS can demonstrate absent or slowed conduction velocities. The needle EMG often remains normal or only with decreased recruitment [70].
Chronic Inflammatory Demyelinating Polyneuropathy
Chronic inflammatory demyelinating polyneuropathy (CIDP) has been reported in patients with solid and liquid tumors including adenocarcinomas, melanoma, and paraproteinemias [71, 72]. Clinically, these patients have slowly progressive sensory changes with large fiber sensory modalities being most affected. CIDP is distinguished from AIDP on the basis of a prolonged and relapsing course, enlargement of nerves, and responsiveness to corticosteroids. Progression tends to be in a length-dependent manner for the sensory changes. The motor abnormalities are typically not as pronounced but are frequently present. The neuropathy may precede the discovery of the neoplasm. Similar to what is seen with acute inflammatory demyelinating polyneuropathy, the electrophysiologic studies demonstrate prolonged or absent F waves, increased distal latencies, abnormal temporal dispersion, and conduction block of motor responses [73].
Paraproteinemic Neuropathies
The prevalence of peripheral neuropathy is ten times higher in patients with paraproteinemias than it is in the general population. Peripheral neuropathy can occur with primary amyloidosis, multiple myeloma, monoclonal gammopathy of unknown significance, Waldenstrom macroglobulinemia, and other rare conditions. Paraproteinemic neuropathies are a heterogeneous group of disorders but with a great degree of overlap.
Patients with primary amyloidosis of the light chain type can develop a devastating peripheral neuropathy. The neuropathy consists of distal and symmetric progressive sensorimotor and autonomic dysfunction. The sensory symptoms are often small fiber predominant with persistent pain. Nerve conduction studies demonstrate decreased amplitude and, at times, mild slowing of conduction velocity consistent with axonal degeneration due to invasion of the nerves by amyloid. EMG can demonstrate increased spontaneous activity and evidence of acute denervation [67, 74].
Peripheral neuropathy can occur in multiple myeloma (MM) in several settings. With typical osteolytic MM, patients can have a mild sensorimotor axonal neuropathy with decreased motor and sensory amplitudes or a purely sensory axonal neuropathy with only sensory involvement. A demyelinating type of primary motor neuropathy can also be seen and resembles AIDP or CIDP as described above. Patients with MM associated with systemic amyloidosis can have prominent pain and small fiber sensory findings with electrophysiologic findings as seen in primary amyloidosis. Patients with atypical osteosclerotic myeloma tend to be younger and less ill. The neuropathy associated with osteosclerotic myeloma is chronic in nature with distal and symmetric sensorimotor changes. This neuropathy also resembles CIDP with a motor predominance and marked slowing in conduction velocities. These patients can develop a clinical syndrome (POEMS syndrome) with organomegaly, endocrinopathy, and skin changes [67, 74].
Patients with monoclonal gammopathy of unknown significance (MGUS) typically present with distal and symmetric chronic and progressive sensorimotor symptoms. Electrophysiologically, the peripheral neuropathy resembles a chronic inflammatory demyelinating polyneuropathy with slow motor nerve conduction velocities and increased distal latencies on NCS. Some MGUS patients have circulating anti-myelin-associated protein (anti-MAG) antibodies directed against peripheral nerve myelin. The majority of patients with anti-MAG antibodies have IgM-kappa monoclonal proteins in their serum [67, 74, 75].
Patients with Waldenstrom macroglobulinemia (WM) can develop symmetric and distal sensorimotor changes and a primarily demyelinating sensorimotor polyneuropathy on electrophysiologic testing. Similar to MGUS, some WM patients have circulating anti-MAG antibodies damaging the myelin and causing increased distal latency and slowed conduction velocity in motor and sensory nerves. Occasionally, WM is associated with an axonal pattern of neuropathy [67, 74, 75].
Anti-CV2
Anti-CV2 antibodies are seen in association with small cell lung cancer and can cause a variety of nervous system maladies including cerebellar degeneration, peripheral neuropathy, and uveitis. In up to 20% of patients, the anti-Hu antibody is also expressed. Patients with anti-CV2 typically have symmetric distal length-dependent sensory loss in all modalities with concomitant motor weakness. Electrophysiologically, a sensory motor axonal polyneuropathy is seen, but at times there are some demyelinating features. NCS demonstrates decreased amplitude of sensory and motor responses with some decrease in velocity of these responses [76].
Anti-Hu
Anti-Hu antibodies are now recognized as a common cause of paraneoplastic neuronopathy that affects the dorsal root ganglia. It is most commonly associated with small cell lung cancer, but is also present with other malignancies. The onset is typically rapid and painful but, unlike classic peripheral neuropathies, this neuronopathy can be asymmetric or non-length dependent in some patients. All sensory modalities can be affected, but the proprioceptive loss can be so profound that patients can have debilitating sensory ataxia. Anti-Hu was once thought to be only a sensory disorder, but evidence shows that some patients have a mixed sensorimotor picture [77, 78]. Electrophysiologic studies demonstrate axonal degeneration with low amplitude or absent sensory action potentials and preserved or abnormal motor responses on NCS. EMG is typically normal but, at times, can show signs of active denervation including fibrillation potentials and positive sharp waves [77, 79].
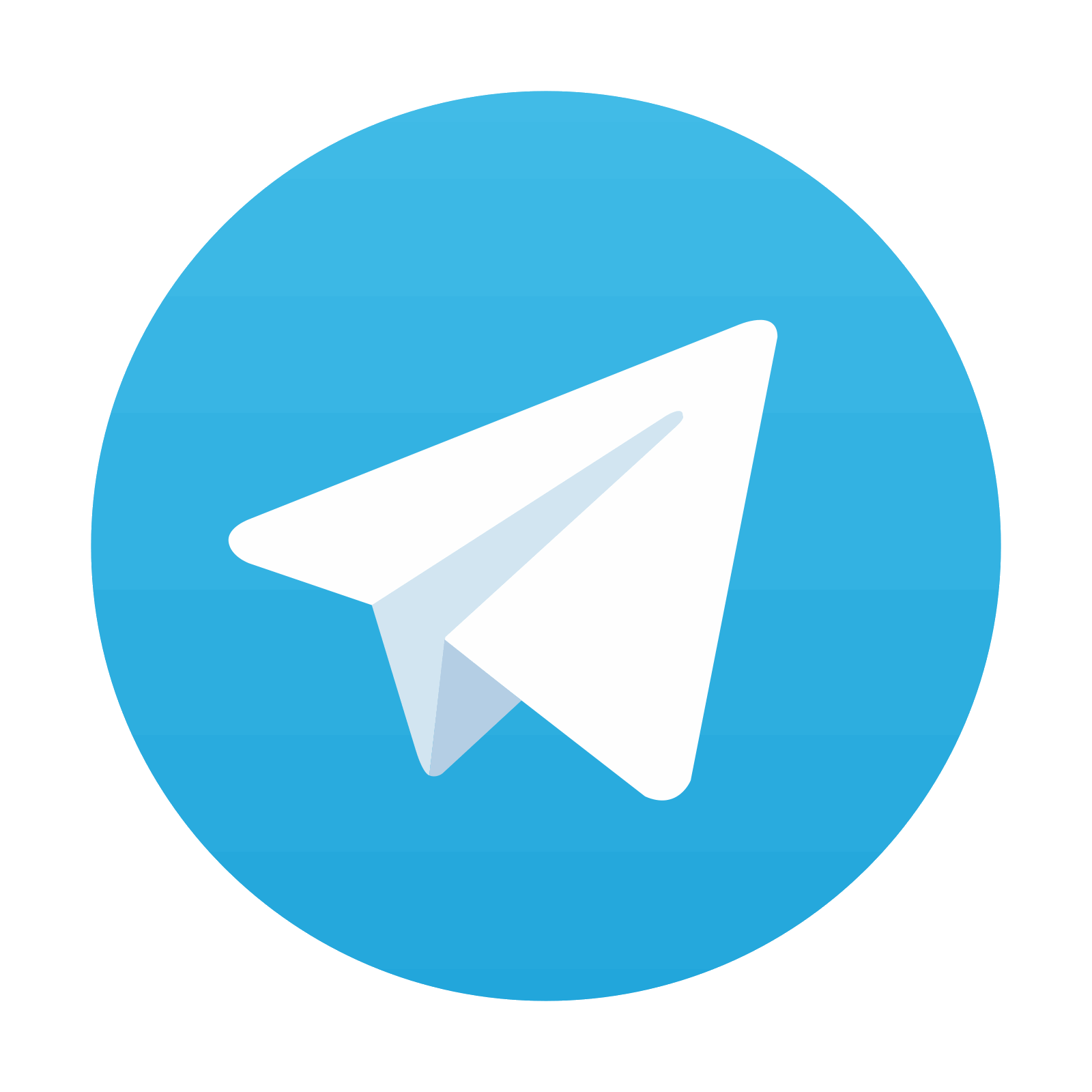
Stay updated, free articles. Join our Telegram channel
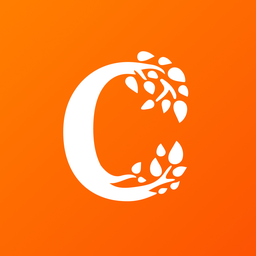
Full access? Get Clinical Tree
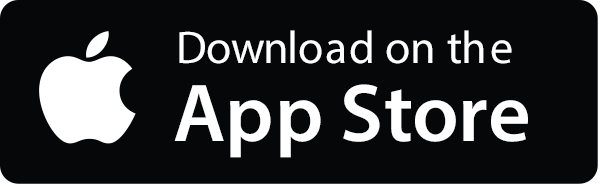
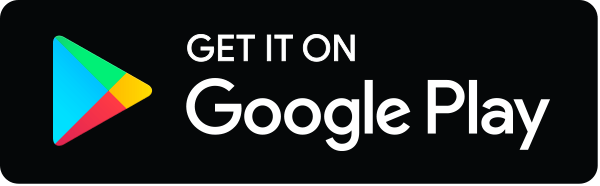