Fig. 28.1
General mechanisms of blood vessel formation. The formation of functional blood vessels can occur by several mechanisms. Three major mechanisms by which blood vessels may form include, Vasculogenesis, Arteriogenesis and Angiogenesis. Vasculogenesis is the process by which new blood vessels form from precursor cells such as hemangioblasts. Arteriogenesis is the process by which functional blood vessels form from small non-functional collateral vessels. Angiogenesis is the process by which new blood vessels form from pre-existing vessels
In contrast to vasculogenesis, arteriogenesis can be described as the formation of functional blood vessels by the activation and expansion of pre-existing small non-functional collateral vessels. Angiogenesis, which will be the major focus of this overview, is the formation of new blood vessels from pre-existing blood vessels. Angiogenesis can be divided into intussusceptive and sprouting angiogenesis. During intussusception, vessels are functionally split by the formation of intra-luminal tissue walls, which result in the formation of higher order branching structures (Nico et al. 2010). In general, this form of vascular remodeling does not require extensive endothelial cell proliferation or extracellular matrix (ECM) remodeling and may occur more often during embryonic development than during postnatal vessel formation. However, examples of intussusceptive angiogenesis have been described in some tumor types such as GBM (Nico et al. 2010). Interestingly, evidence now suggests that recovery of some tumor blood vessels following anti-angiogenic treatment may occur by intussusceptive rather than sprouting angiogenesis (Nico et al. 2010). Given that intussusception does not require significant cellular proliferation, treatments with conventional anti-angiogenic drugs might have little impact on tumor perfusion under these circumstances.
Sprouting angiogenesis on the other hand is distinct from that of intussusception, as specialized endothelial tip cells are activated by a gradient of angiogenic stimuli to induce remodeling of the local ECM and directional invasion and migration into the surrounding tissue. Interestingly, the formation of the activated endothelial tip cells has been suggested to be controlled at least in part, by Notch/Dll4 signaling (Hellstrom et al. 2007). Studies have shown that Notch/Dll4 signaling may act to regulate the formation of endothelial tip cells and reduce sprouting in vivo. These activated endothelial cells along with their associated endothelial stalk cells are thought to organize into a solid cord of cells, undergo a complex process of lumen formation or canularization and finally, recruit supporting accessory cells to stabilize the newly forming blood vessel. This continuum of events within the angiogenic cascade can be organized into three interconnected phases (Fig. 28.2) including an initiation phase, an invasive phase and a maturation phase. The complex cellular and molecular mechanisms involved in the formation of functional blood vessels require fine-tuning and cooperation among numerous cell types and a wide variety of molecular regulators. For example, while Vascular Endothelial Cell Growth Factor (VEGF) is well known to stimulate vessel formation, signaling mechanisms have evolved to limit excessive vascular growth in order to optimize vessel function to provide adequate tissue perfusion. When these finely tuned mechanisms go out of balance, it can lead to either uncontrolled vessel formation and/or the formation of non-productive vessels. To this end, studies have shown that in addition to stimulating angiogenesis, VEGF can induce expression of regulators that act to limit new blood vessel formation. An example of this negative feed back system involves the VEGF induced Notch/DlI4 signaling loop which may limit the number of endothelial tip cells formed during angiogenesis (Hellstrom et al. 2007). Moreover, VEGF may also limit functional blood vessel formation by altering PDGFβ signaling in smooth muscle cells and the subsequent recruitment of pericytes to stabilize the developing vessel (Greenberg et al. 2008). Thus, as with most signaling pathways that govern complex biological processes, the tissue specific repertoire of regulatory factors and the extent of the activation of negative feed back loops are critical for productive vessel formation.
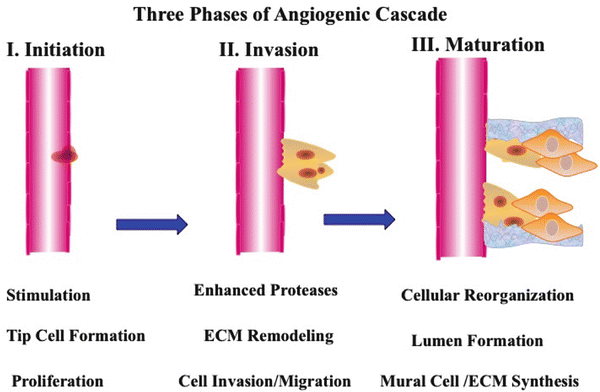
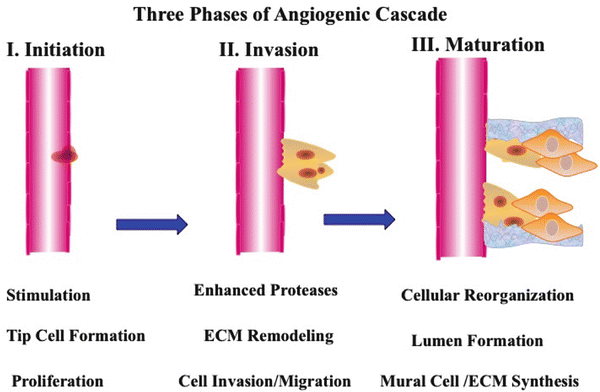
Fig. 28.2
The angiogenic cascade. Angiogenesis or the process by which new blood vessels form from pre-existing vessels requires a highly integrated set of molecular and cellular events. The interconnected cascade of events that govern angiogenesis can be organized into three stages. The initiation phase can be characterized by growth factor stimulated formation of tip cells and endothelial cell proliferation. The invasive phase of angiogenesis can be characterized by enhanced expression of proteolytic enzymes, localized extracellular matrix (ECM) remodeling and cellular invasion and migration. Cellular re-organization, lumen formation, recruitment of mural cells and synthesis of new ECM components to provide support for the newly forming vessel characterize the maturation phase
Additional layers of complexity involved with angiogenesis may be observed when considering tissue specific angiogenesis. For example, distinct organ microenvironments may require unique combinations of the various classes of regulatory components needed for successful completion of the angiogenic cascade. New evidence is accumulating that tissue specific alteration in the expression of small noncoding RNAs called miRNAs may play an important role in fine-tuning the expression of crucial angiogenesis regulatory molecules. In fact, investigators have identified several miRNAs, which regulate angiogenesis. Interestingly, the miRNA-296 was increased in association with brain tumor endothelial cells as compared to normal brain endothelial cells (Wurdinger et al. 2008). Moreover, miRNA-132 has also been shown to be upregulated in tumor endothelial cells as compared to normal endothelial cells and has been suggested to play a critical role in promoting the angiogenic switch (Anand et al. 2010). Given these observations, it would not be surprising that pathological angiogenesis occurring within one particular organ may not be the same as that occurring in a different organ system. This emerging idea needs to be considered as more emphasis is being placed on the broad use of anti-angiogenic drugs for the treatment of malignant tumors. Here, we will focus on the cellular and molecular mechanisms that regulate pathological angiogenesis with an emphasis on vessel formation within the unique microenvironment of the central nervous system.
Cellular and Molecular Regulators of the Angiogenic Cascade
While it is likely that tissue specific mechanisms exist to control angiogenesis within a given tissue microenvironment, the overall angiogenic cascade can be organized into a continuum of three interconnected steps (Fig. 28.2). We will briefly discuss several well-characterized examples of the major cellular and molecular regulators of angiogenesis as they relate to the functional steps that occur during new blood vessel formation. While it has long been appreciated that endothelial and mural cells such as vascular smooth muscle cells and pericytes play active roles in physiological and pathological angiogenesis, new evidence is providing critical insight into the functional roles of numerous additional cell types in the angiogenic cascade. Studies have provided compelling evidence that cell types as diverse as fibroblast, tumor cells, bone-marrow derived progenitor cells as well as inflammatory cells such as macrophages and mast cells all contribute to the formation of new blood vessels (Contois et al. 2009). These important findings are crucial to our overall mechanistic understanding of angiogenesis, as these diverse cell types are known to represent many of the major tissue specific sources of miRNAs that may ultimately contribute to controlling the relative expression of pro and anti-angiogenic factors. Thus, it is interesting to note that these accessory cells may well represent important new therapeutic targets to help control pathological angiogenesis.
While tumor cells are well known to express many soluble pro-angiogenic growth factors, cytokines and chemokines such as VEGF, PDGF, FGF, IL-8, and SDF-1, a number of inflammatory cells such as mast cell and macrophages also express many similar pro-angiogenic molecules. In addition, distinct cell types may also contribute to the generation of endogenous angiogenesis inhibitors formed as fragments of larger molecules such as Angiostatin, PEX, Endostatin, and several NC1 domains of collagen type-IV (Contois et al. 2009). While the molecular mechanisms by which these inhibitors function in vivo is not completely understood, the relative levels of these factors in relationship to overall levels of pro-angiogenic molecules helps to dictate whether or not the angiogenic switch is activated and whether new blood vessels begin to form. Therefore, as it can be appreciated from this brief summary, many diverse cell types beyond endothelial cells play critical roles in angiogenesis.
In addition to the expression of growth factor, cytokines, chemokines and their respective cell surface receptors, other classes of regulatory molecules also play crucial roles in angiogenesis including several different types of adhesion molecules. For example, during the initiation phase, pro-angiogenic stimulation by growth factors can lead to alterations in endothelial cell-cell and cell-ECM interactions. Studies have suggested that stimulation by growth factors such as VEGF can alter the functional localization and activity of Vascular Endothelial Cadherin (VE-Cadherin), a well-characterized cell-cell adhesion molecule known to play a role in angiogenesis (Dejana et al. 2008). This altered localization and functional activity can lead to disruption of endothelial cell-cell contacts thereby promoting vascular leakage of serum proteins that contributes to the formation of a modified ECM and enhance cellular invasion and migration. This modified ECM may also provide selective homing signals to facilitate localization of additional angiogenic accessory cells. Growth factor stimulation can also enhance the expression and activation of a class of transmembrane cell adhesion receptors termed integrins. An expanding group of integrins including α1β1, α2β1, α4β1, α5β1, α6β4 αvβ3, αvβ5 and αvβ8 are all though to play roles in regulating angiogenesis (Contois et al. 2009). It is important to point out that these alterations in adhesion are not restricted solely to endothelial cells but may occur in other cells known to play roles in neovascularization. This altered adhesive activity can in turn contribute to changes in expression of proteolytic enzymes, another critical group of angiogenic regulatory molecules.
Importantly, integrin mediated signaling within a variety of cell types such as endothelial cells; fibroblasts and tumor cells can result in enhanced expression and activity of proteolytic enzymes such as matrix metalloproteinases (MMPs) and serine proteases (Contois et al. 2009). Release of various chemoattractants can act to recruit additional cell types such as macrophages, mast cells and bone marrow derived myeloid cells, which collectively contribute to overall levels of proteolytic enzymes at sites of new vessel growth. This localized increase in proteolytic activity can in turn further modify the vascular ECM. This newly remodeled vascular ECM has been shown to play an active role in facilitating angiogenesis by mechanisms as diverse as enhancing the release of matrix bound growth factors such as VEGF, to exposing unique matrix immobilized cryptic ECM epitopes (MICEE) to facilitate localized integrin signaling events that contribute to adhesion, migration and proliferation (Contois et al. 2009).
While growth factors, cell adhesion molecules, ECM proteins, proteolytic enzymes and many other factors all contribute to new blood vessel development, they do not function in isolation, but rather work cooperatively within an integrated network to allow productive angiogenesis to occur. During the initiation phase of angiogenesis, the balance between pro and anti-angiogenic regulatory molecules become shifted in favor of angiogenic stimulation, resulting in a significant increase in the relative expression of a number of soluble pro-angiogenic factors such as VEGF, FGF2, IGF-1, IL-8 and SDF-1. The relative levels of these angiogenic factors may be fine tuned by miRNAs. Conversely, the expression of certain miRNAs can be governed by angiogenic growth factors. For example, VEGF and FGF2 have recently been shown to mediate induction of miRNA-132 in endothelial cells (Anand et al. 2010). As mentioned above, this increased expression of pro angiogenic growth factors can originate from a variety of cell types within the microenvironment including tumor cells, inflammatory infiltrates, cancer associated fibroblasts as well as numerous bone marrow derived myeloid cells. Secreted pro-angiogenic molecules can bind their respective cell surface receptors on endothelial cells to activate a cascade of down stream signaling networks such as P13K/Akt pathways that ultimately lead to enhanced cellular proliferation, altered expression and activation of cell adhesion molecules and enhanced expression of proteolytic enzymes (Jiang and Lui 2009). Interestingly, recent evidence suggests that miRNA-126 may specifically regulate down stream signaling associated with both MAP/Erk and PI3k/Akt signaling pathways during new vessel formation (Wang et al. 2008). In fact, the ability of miRNA-126 to modulate angiogenesis was shown to be associated in part, with its ability to repress expression of the sprouty-related protein SPRED1, which is thought to modulate MAP/Erk signaling (Wang et al. 2008). Interestingly, members of the Sprouty family, which are thought to negatively regulate MAP/Erk signaling, may also regulate angiogenesis. For example, recent studies indicate that overexpression of Sprouty-4 may inhibit embryonic vascular sprouting, while Sprouty-1 may inhibit endothelial cells proliferation in part by altering levels of the cyclin dependent kinase inhibitors P21 and P27 (Lee et al. 2001, 2010). In addition to impacting the MAP/Erk pathway, miRNA-126 has also been shown to modulate the PI3k/Akt pathway by altering the expression of the PI3kinase regulatory subunit p85β (Wang et al. 2008). Intriguing new evidence suggests that growth factor-mediated upregulation of miRNA-132 in endothelial cells may repress expression of p120RASGAP, which results in increased RAS activity and enhanced endothelial cell proliferation and angiogenesis in vivo (Anand et al. 2010). In fact, antagomers directed to block miRNA-132 were shown to inhibit angiogenesis and tumor growth in multiple models (Anand et al. 2010). Finally, pro-angiogenic stimulation can be further enhanced by growth factors stimulation of additional accessory cells, which collectively add to the overall proliferative loop within the local microenvironment.
The initiation phase of angiogenesis is followed closely by the invasive phase. Proteolytic remodeling of the vascular basement membrane as well as the interstitial matrix can result in structural changes within the ECM. These changes may contribute to the release of matrix bound growth factors to enhance cellular proliferation and migration. In addition, biomechanical alterations in the structural integrity of ECM molecules such as collagen and laminin may specifically expose cryptic regulatory sites that are recognized by cell adhesion receptors such as integrins (Xu et al. 2001; Akula et al. 2007). These new receptor ligand interactions may allow initiation of distinct signaling cascades that are largely restricted to sites of matrix remodeling. Recent evidence has indicated that cellular interactions with these newly exposed cryptic sites within the matrix may regulate proliferation, survival and facilitate invasion and migration during angiogenesis and other invasive cellular processes (Xu et al. 2001; Akula et al. 2007). In fact, antibodies directed to these cryptic sites have been shown to inhibit angiogenesis, tumor growth and metastasis in vivo (Xu et al. 2001; Akula et al. 2007). These studies have recently lead to the translation of this new approach into a human clinical trial. In particular, a Phase I clinical trial using a novel Mab termed TRC093 directed to a cryptic collagen epitope has been completed (Robert et al. 2010). This novel approach of targeting highly selective cryptic sites within the ECM, rather than direct targeting of individual vascular cells, resulted in little if any toxicity and importantly, evidence of antitumor activity along with reduced levels of circulating VEGF was observed (Robert et al. 2010).
In contrast to promoting angiogenesis, proteolytic enzymes may also lead to limiting new blood vessel formation. For example, protease mediated degradation of ECM proteins may result in release of inhibitory fragments of collagen such as endostatin and several NC1 domains of collagen type-IV (Kessenbrock et al. 2010). It is interesting to point out that certain MMPs such as MMP-8 may be associated with reduced tumor progression, which might be due to multiple mechanisms such as to excessive proteolysis leading to inactivation of cell adhesion receptors, degradation of angiogenic growth factors and elevated release of endogenous ECM-derived angiogenesis inhibitors (Kessenbrock et al. 2010). Collectively, these studies again illustrate the fine balance that is needed between pro and anti-angiogenic signaling events for productive angiogenesis to occur.
During the maturation phase, endothelial cells begin to reorganize into cords of cells. These reorganized endothelial cells exhibit altered phenotypic characteristics by establishing new cell-cell interactions mediated by cell adhesion molecules such as cadherins. New signaling cascades facilitated by both cell-cell as well as integrin mediated interaction with the surrounding ECM contributes to the formation of a functional lumen by a process termed canularization. While relatively little is known concerning the molecular mechanisms regulating canularization, recent studies have implicated diverse molecules such integrin α2β1, MMPs, and VE-Cadherin in lumen formation (Davis et al. 2007). In addition to lumen formation during vessel maturation, the measured release of growth factors and chemokines are thought to help recruit mural cells, pericytes and potentially, bone marrow derived progenitor cells to support the newly forming vessel. The newly sequestered supporting cells also contribute to the expression of ECM molecules which help construct new vascular basement membranes, which when taken together with the accessory cells, provides mechanical support for the maturing vessel. As can be appreciated from this brief overview of the general steps in the angiogenic cascade, it would not be surprising that numerous differences exist between physiological and pathological angiogenesis.
Physiological vs. Pathological Angiogenesis
It has been known for decades that distinct differences exist between normal blood vessels and blood vessels that form during pathological processes such as tumor growth. One of the most obvious differences involves a striking alteration in morphology. In contrast to quiescent blood vessels, tumor blood vessel are often characterized as being highly proliferative, leaky, tortuous vessels with altered basement membranes and reduced numbers of supporting cells. Studies have shown that tumor endothelial cells may exhibit altered expression of a variety of regulatory molecules that contribute to the unique phenotype of tumor blood vessels and this may provide important molecular insight into pathological angiogenesis. For example, studies have shown elevated expression of efflux pump proteins such as ABCG2 in GBM blood vessels as well as increased levels of apoptosis regulators such survivin (Zhang et al. 2003). Moreover, following laser capture micro-dissection, isolated GBM endothelial cells exhibited enhanced resistance to cytotoxic drugs, elevated levels of growth factors and cytokines such VEGF, IL-8 and CXCL12/SDF1, and altered expression of miRNAs that regulate angiogenesis such as miRNA-296 (Wurdinger et al. 2008). Interestingly, endothelial cells derived from multiple tumor types were shown to express elevated levels of miRNA-132 as compared to quiescent endothelial cells (Anand et al. 2010). Finally, GBM endothelial cells exhibited increased migratory capacity as compared to their normal counterparts. All of these factors and many others have been shown to impact new blood vessel development and thus may contribute to angiogenesis in a tissue specific manner.
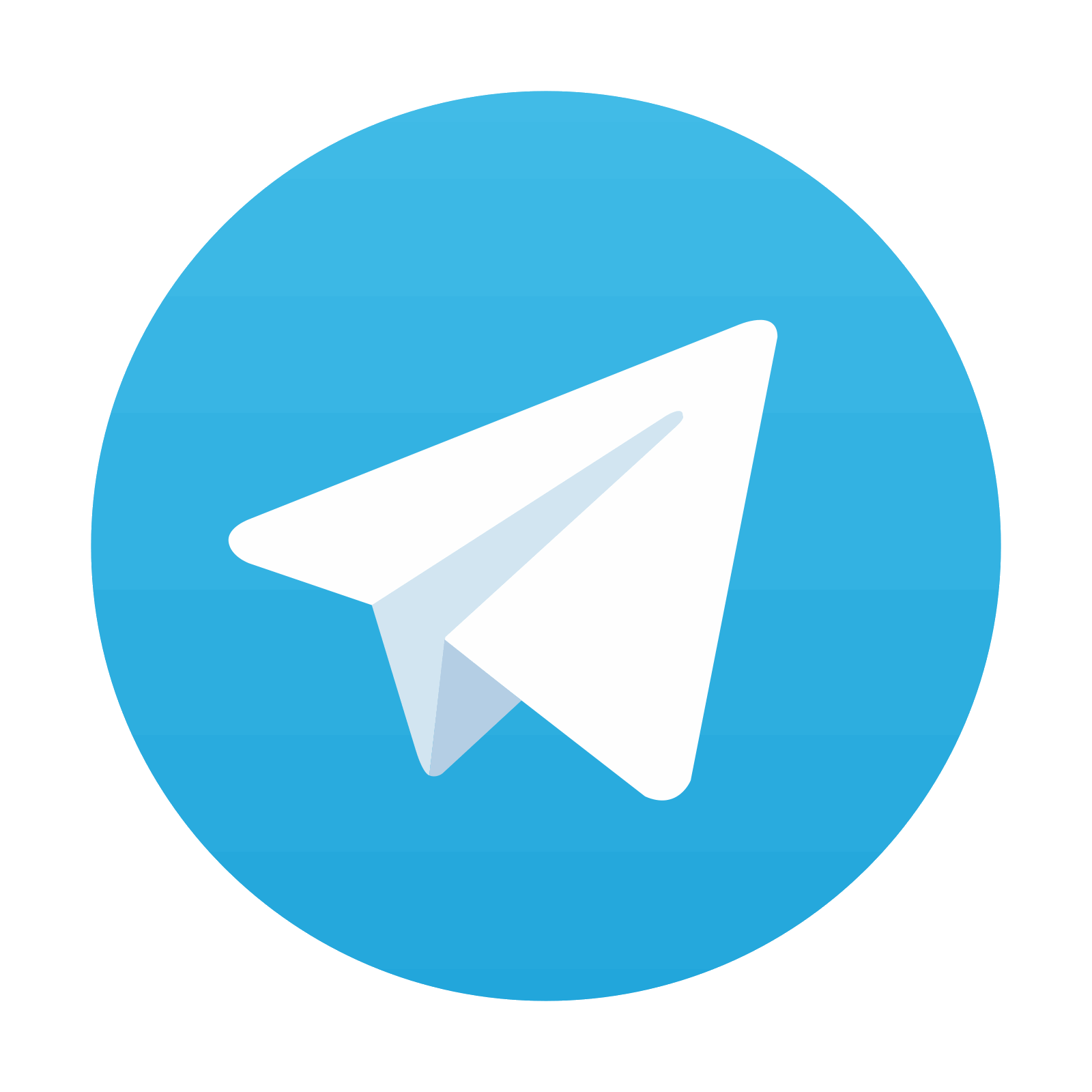
Stay updated, free articles. Join our Telegram channel
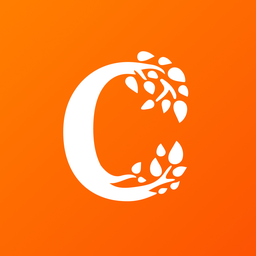
Full access? Get Clinical Tree
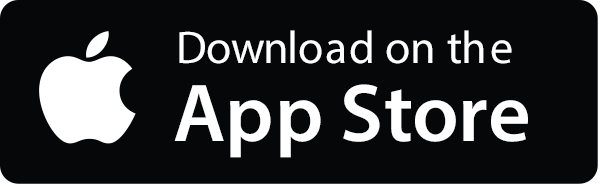
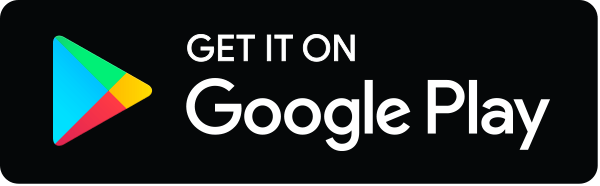