, Jean Paul G. Vonsattel2, Helmut Heinsen3, 4 and Horst-Werner Korf5
(1)
Dr. Senckenbergisches Chronomedizinisches Institut, Goethe University Frankfurt, Frankfurt, Germany
(2)
Medical Center Neurological Institute, Columbia University, New York, NY, USA
(3)
Division Psychiatic Clinic Morphological Brain Research Unit, Julius Maximilians University Würzburg, Würzburg, Germany
(4)
University of Sao Paulo Medical School, Sao Paulo, Brazil
(5)
Dr. Senckenbergisches Chronomedizinisches Institut, Goethe University, Frankfurt, Frankfurt, Germany
10.1 Morphological Alterations of Vulnerable Nerve Cells in Huntington’s Disease (HD)
Except for the atrophic neurons in the striatum referred to as neostriatal dark neurons (NDN) (see Sect. 2.2), no consistent pathognomonic alterations of vulnerable brain nerve cells have been reported during more than hundred years of morphological HD research. In particular, despite recent careful and systematical investigations of possibly affected brain regions, no nerve cells have been observed in HD brains which display morphological features associated with the occurrence of classical apoptosis (i.e., chromatin condensation, nuclear fragmentation, apoptotic bodies) (Graeber and Moran 2002; Rüb et al. 2013a). Classical apoptosis is regarded as a specific form of programmed cell death and is defined as a series of stereotypical, biochemical, and morphological alterations leading to nerve cell demise. Classical apoptosis regulates the balance between proliferation and differentiation in the course of brain development and during optimization of adult nerve cell functions. Although it has been implicated in the pathogenesis of neurodegenerative diseases, classical apoptosis cannot account for all cell death phenotypes occurring in these diseases. Therefore, other forms of nerve cell death mechanisms may occur in human diseases affecting the terminally differentiated, postmitotic nerve cells. The absence of apoptotic nerve cells in HD brains supports this point of view and conforms to recent HD studies suggesting that the molecular mechanisms of classical apoptosis do not play a significant role for neurodegeneration occurring in HD patients or in transgenic HD models (Gil and Rego 2008; Graeber and Moran 2002; Pattison et al. 2006; Rüb et al. 2013a).
10.2 Detection of Ballooned or Chromatolytic Nerve Cells
The observation and first description of so-called ballooned or chromatolytic nerve cells in the affected cerebellum of HD patients has changed this situation and may provide an important gateway to better understand the pathogenesis of nerve cell death in HD (Rüb et al. 2013a). Along with shrunken nerve cells, ballooned nerve cells represent the vast majority of remaining cerebellar nerve cells of HD patients and have also been abundantly observed in the degenerated thalamus and brainstem of clinically diagnosed and genetically confirmed HD patients (Figs. 5.3, 5.4, and 5.5) (Rüb et al. 2015; Rüb et al. 2013a) (see Sect. 5.2). Ballooned nerve cells cannot be regarded as disease-specific or pathognomonic neuropathological features of HD. They may also occur in large quantities at multiple brain sites in human tauopathies (i.e., Alzheimer’s disease, AD; argyrophilic grains disease, AGD; corticobasal degeneration, CBD; progressive supranuclear palsy, PSP), but their brain distribution pattern in these human tauopathies is different from that observable in HD (Dickson 1999; Fujino et al. 2004; Grinberg and Heinsen 2009; Kato et al. 1992; Rüb et al. 2013a; Stover and Watts 2001). The rounded and massively enlarged cytoplasm of ballooned neurons harbors a homogeneous central basophilic substance. Their Nissl substance, lipofuscin granules, and flattened nucleus are displaced along the cytoplasmic membrane and mimic central chromatolysis. This massive enlargement of the perikarya of ballooned neurons is linked to structural damage of their axons and/or functional impairment of intra–axonal transport mechanisms (see Sect. 5.2) (Figs. 5.3, 5.4, and 5.5) (Fujino et al. 2004; Kato et al. 1992; Rüb et al. 2013a).
Recent immunocytochemical studies have shown that axonal pathologies in the brains of HD patients along with the occurrence of calbindin-immunoreactive torpedo-like inclusions in the axons of cerebellar Purkinje cells also include the presence of axonal aggregates of the disease protein huntingtin (Htt) in additional gray and white matter brain components of HD patients (e.g., neostriatum, cerebral neocortex, internal capsule, cerebral peduncle), as well as axonal inclusions in brainstem fiber tracts (see Sects. 5.2 and 9.2) (Figs. 5.2, 9.3, 9.4, and 9.5) (Atkin and Paulson 2014; Becher et al. 1998; Borrell-Pagès et al. 2006; Finkbeiner and Mitra 2008; Gil and Rego 2008; Gourfinkel-An et al. 1998; Gunawardena and Goldstein 2005; Gutekunst et al. 1999; Imarisio et al. 2008; Kuemmerle et al. 1999; Labbadia and Morimoto 2013; Li and Conforti 2013; Li and Li 2011; Maat-Schieman et al. 2007; Ortega et al. 2007; Paul 2008; Rüb et al. 2014a; Schulte and Littleton 2011; Sieradzan et al. 1999; Valera et al. 2005; Walker 2007b; Wooten et al. 2006). Considering the occurrence of these diverse axonal pathologies in the brains of HD patients and the well-known association between axonal damage and the transition of affected nerve cells toward ballooned nerve cells, it appears conceivable that elucidation of the significance and role of the HD-associated axonal pathology in the pathogenesis of ballooned nerve cells (1) may represent a new gateway to the pathological process of HD, (2) can provide new and important insights into the pathological mechanisms of nerve cell demise in HD, and (3) may lead to the identification of early and crucial steps in the cascades of pathological events that via the intermediate stage of nerve cell swelling eventually lead to destabilization, shrinkage, and death of susceptible nerve cells. These cascades of pathological events preceding demise of nerve cells may include the following: (1) An initial and yet unknown causative pathological condition or event in diseased nerve cells that initiates the pathological cascades and sooner or later leads to axonal dysfunctions and/or damage, swelling or ballooning of affected nerve cells, and displacement and compression of their cellular organelles. (2) Loss of the balance between pathological and protective mechanisms (i.e., expression of heat shock proteins, HSP) competing and interfering in these vulnerable nerve cells. (3) Failure and exhaustion of protective mechanisms in ballooned nerve cells. (4) Loss of nerve cell organelles (i.e., mitochondria, Nissl substance) and severe shrinkage of the nucleus and cytoplasm of nerve cells. (5) Ultimate degeneration and demise of exhausted nerve cells leaving behind structurally stable lipofuscin granules in the neuropil which represent reliable markers of the former position of demised nerve cells (Braak et al. 2003b; Rüb et al. 2001, 2003a, 2004a, 2008a, b, 2009, 2013b; Scherzed et al. 2012).
Mitochondria are well known for their role in cellular energy supply via ATP generation through oxidative phosphorylation. Since their cellular functions are also needed throughout the axon of nerve cells, the functional integrity, unimpaired intracellular trafficking, as well as positioning of mitochondria in accordance to local energy requirements are therefore pivotal for the survival of nerve cells. The traditional view of mitochondria of nerve cells as powerhouses quietly lingering around and resting in the cytosol of cells has been stepwise replaced in the past by the perspective of a dynamic intraneuronal mitochondrial trafficking network that physically connects remote cellular compartments (such as neuronal synapses) to the soma of nerve cells and is thus involved in major cellular functions. After biogenesis in the cell body of nerve cells, mitochondria together with other cellular organelles and newly synthesized protein complexes are delivered into the axon, loaded onto molecular kinesin motors, and anterogradely transported along microtubule tracks to the synaptic terminal (Fig. 8.2) (De Vos et al. 2008; Gunawardena and Goldstein 2005; Li and Conforti 2013; Millecamps and Julien 2013; Ortega et al. 2007). While some of the anterogradely transported mitochondria move persistently over long distances (tens to hundreds of micrometers), others appear anchored or remain stationary in the axon according to specific local energy demands. Since accumulating oxidative damage impairs the functions of mitochondria during the period of their service in the axon, aged and impaired mitochondria are returned via molecular dynein motors and retrograde transport mechanisms to the nerve cell body for degradation (see Sect. 8.1) (De Vos et al. 2008; Gunawardena and Goldstein 2005; Li and Conforti 2013; Millecamps and Julien 2013; Ortega et al. 2007).
The axonal pathologies observed in the brains of HD patients most likely are detrimental to axonal transport mechanisms, which in turn result in disruption of adequate mitochondrial trafficking along axons and deficient neuronal energy production and supply (see Sects. 8.1, 9.1, 9.2, and 10.2) (Borrell-Pagès et al. 2006; Davies et al. 2007; De Vos et al. 2008; Finkbeiner and Mitra 2008; Gil and Rego 2008; Gunawardena and Goldstein 2005; Imarisio et al. 2008; Labbadia and Morimoto 2013; Li and Conforti 2013; Li and Li 2011; Millecamps and Julien 2013; Oliveira 2010; Ortega et al. 2007; Pandey et al. 2010; Rüb et al. 2013a, 2014a; Schulte and Littleton 2011; Walker 2007a, b). Since failure of mitochondrial energy production is associated with a variety of late-onset neurodegenerative diseases including HD, the involvement of mitochondria and their dysfunction in the pathogenic context of neurodegenerative disorders are now generally accepted. Although additional impairments may contribute to mitochondrial dysfunction and decreased energy production in HD (Borrell-Pagès et al. 2006; Davies et al. 2007; De Vos et al. 2008; Finkbeiner and Mitra 2008; Gil and Rego 2008; Gunawardena and Goldstein 2005; Imarisio et al. 2008; Labbadia and Morimoto 2013; Li and Conforti 2013; Li and Li 2011; Millecamps and Julien 2013; Oliveira 2010; Ortega et al. 2007; Pandey et al. 2010; Rüb et al. 2013a, 2014a; Schulte and Littleton 2011; Walker 2007a, b), damage to mitochondrial trafficking most likely leads to a displacement of these neuronal powerhouses, is detrimental to a timely ATP production and energy supply in accordance to the local demands of specific nerve cell sites, and thus plays a significant role in the pathogenesis of neuronal dysfunction and death during HD. Such impairments of mitochondrial transport not only provide suitable explanations for the disturbed energy situation described in HD patients, but may also cause an accumulation of these essential organelles in the cell body of affected nerve cells, which provokes the massive enlargement of their cytoplasm ultimately giving shape to ballooned or chromatolytic nerve cells. Furthermore mitochondrial dysfunction, which reduces ATP production and energy metabolism, initiates a vicious circle that ultimately leads to further deficits of axonal transport processes and to structural damage of axons (see Sect. 8.1) (Borrell-Pagès et al. 2006; Davies et al. 2007; De Vos et al. 2008; Finkbeiner and Mitra 2008; Gil and Rego 2008; Gunawardena and Goldstein 2005; Imarisio et al. 2008; Labbadia and Morimoto 2013; Li and Conforti 2013; Li and Li 2011; Millecamps and Julien 2013; Oliveira 2010; Ortega et al. 2007; Pandey et al. 2010; Rüb et al. 2013a, 2014a; Schulte and Littleton 2011; Walker 2007a, b).
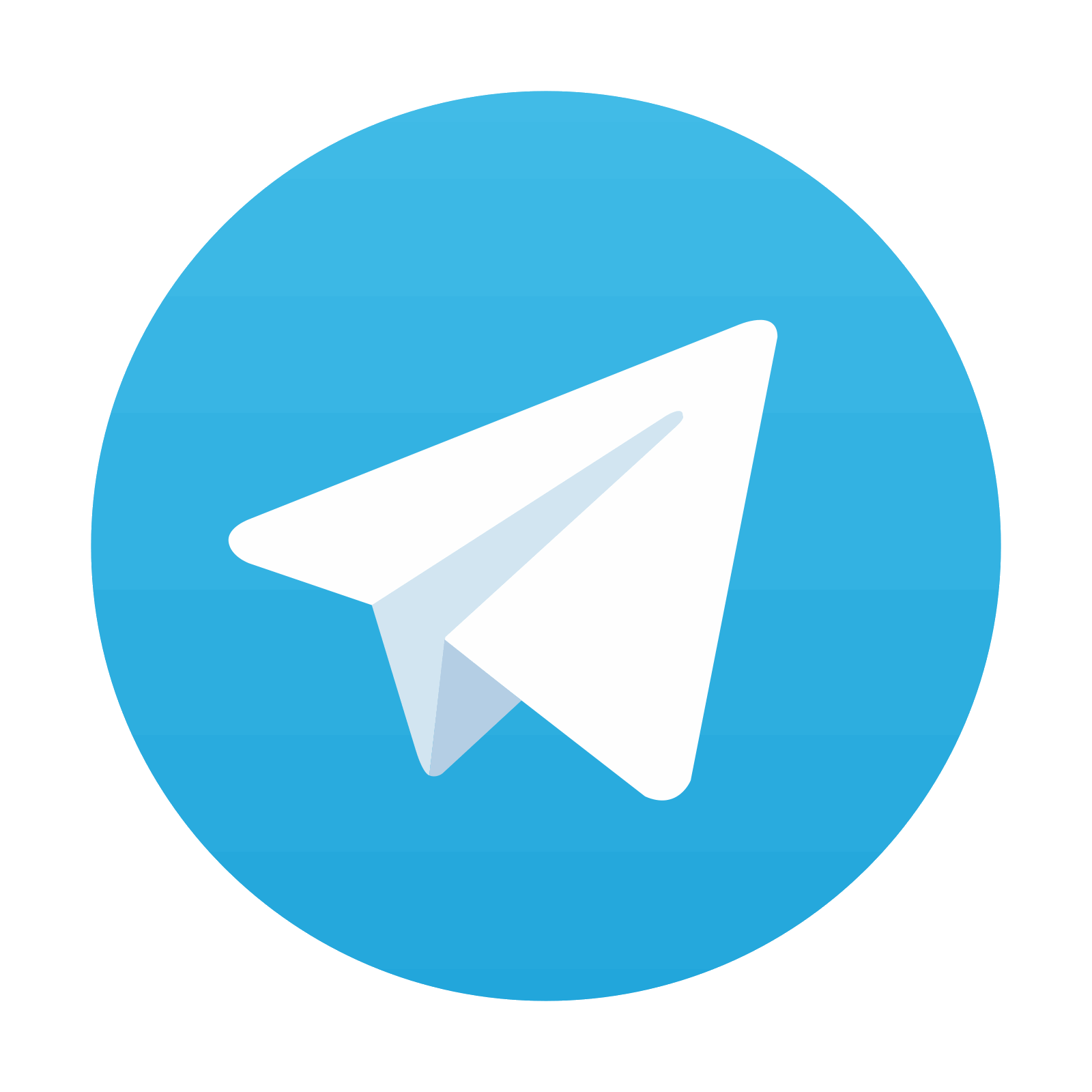
Stay updated, free articles. Join our Telegram channel
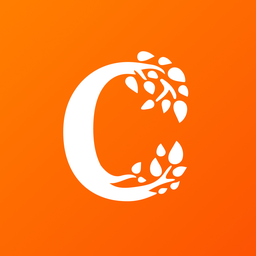
Full access? Get Clinical Tree
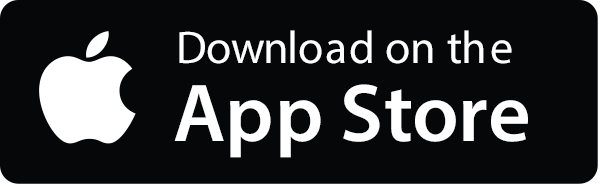
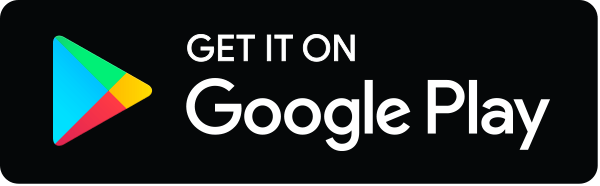