Fig. 29.1
Estimated ED visits for sports-related concussions for ages 8–13 (dotted line) and ages 14–19 (dashed line) and participation for ages 7–17 (solid line) in the top five organized team sports (football, basketball, baseball, soccer, and hockey) from 1997 to 2007 (participation data restricted to 7–17 because of NSGA constraints). Reproduced with permission from Pediatrics, Vol. 126, Page(s) e550–e556, Copyright 2010 by the AAP
In 2010, over 7.6 million students participated in high school sports. While concussion risk is obvious in some sports, like football and ice hockey, the risk may be less clear in “noncontact” sports, like baseball. Concussion rates in all sports appear to be higher during competitions than during practices. Multiple studies have found the majority of concussions to occur during games, despite spending much more time in practice. Additionally, the majority of sports concussions occur in children who have not had prior concussion, with only 10–15 % of reported concussions occurring in children who have already had at least one [13]. Having one concussion approximately doubles the risk of having a second concussion [82]. In gender comparable sports (i.e., soccer, basketball, baseball/softball), girls had roughly double the risk of concussion, both new and recurrent [13, 46].
Concussions are much more likely to result from player-player contact (~70 %) than player-playing surface contact (i.e., collision rather than falls). In sports with extra equipment (e.g., baseball bats/balls, lacrosse sticks/balls, hockey sticks/pucks), player-equipment contact is a frequent cause of concussion as well. Rates are highest in full-contact sports (American football, ice hockey, lacrosse), but still occur at significant rates in minimal contact when compared to noncontact sports [46, 82].
Given the popularity of the sport and the frequency of concussions within it, special attention has been paid to the epidemiology of concussion in American football [27]. Multiple studies have attempted to determine the incidence of concussion in this group, with estimates ranging from 5 to 20 % annually in high school football and 4–5 % annually in college football, accounting for anywhere between 90,000 and 290,000 concussions every year. Given the frequency of high impacts, one study found that nearly 15 % of those athletes who suffered from one concussion sustained a second injury during the same season. The athletes who played defensive back, offensive line, running back, or linebacker positions were the most frequently injured [27, 46]. In football more than any other sports, prior concussion is a risk for recurrent concussion, leading to a two to fourfold increase in risk.
Pathophysiology of Concussion
Biomechanics of Injury
Acceleration/deceleration injuries, rather than direct blows to the head, are the most frequently cause of concussions [64]. When a force is applied to the body or to the head itself, the brain experiences these forces as angular rotation rather than translational (Figs. 29.2 and 29.3). The spinal cord and the brain stem are generally fixed in space by the confines of the spinal column and attachments at the base of the skull (e.g., vessels, cranial nerves). The upper midbrain, thalamus, and cerebral hemispheres are suspended on the brain stem within the skull, surrounded by fluid and connective tissue. These structures are not fixed within this space and are free to move within the skull to varying degrees. The cerebrospinal fluid around the brain acts as somewhat of an inertial damper, redirecting forces that are transmitted to the skull around the brain rather than through it. But it can only dampen so much force, and what force is applied causes the brain to rotate around its more fixed and deep structures. The brief loss or alteration of consciousness associated with concussion is likely an effect of these forces acting on the junction of the upper midbrain and thalamus, leading to a transient disruption in the functioning of the reticular neurons that maintain alertness [76].



Fig. 29.2
Head motion injuries. Impact can cause local contact effects, but two additional effects contribute to the lesions observed clinically. Because of its inertial properties, the brain slides relative to the inner skull surface, and cortical vessels connecting the brain to the dural membrane may tear. Additionally, the inertial loading delivered to the brain, coupled with its soft material properties, leads to deformation of the brain contents. This deformation is visualized with a superimposed grid pattern that deforms from the acceleration imparted during impact (shown as an arrow). This figure was published in Youmans Neurological Surgery, Vol. 4, David F. Meaney, Stephen E. Olvey, and Thomas A. Gennarelli, Chapter 324 Biomechanical Basis of Traumatic Brain Injury, p. 3280, Copyright Elsevier, 2011

Fig. 29.3
Types of head acceleration . Translational acceleration will move the head in a linear path. Alternatively, rotational acceleration will induce rotation about the head’s center of mass, which is located approximately in the pineal region. It is rare to find instances of pure translational or rotational acceleration in “real-world” injury situations. Rather, angular motion is more common. The angular motion leads to combined translational and rotational acceleration, thereby creating injury patterns that arise from both accelerations types. This figure was published in Youmans Neurological Surgery, Vol. 4, David F. Meaney, Stephen E. Olvey, and Thomas A. Gennarelli, Chapter 324 Biomechanical Basis of Traumatic Brain Injury, p. 3281, Copyright Elsevier, 2011
Forces applied to the brain become angular rotational forces due to the structures that constrain the base of the brain [5, 6]. White matter, likely due to the myelin sheaths around axons , is stiffer than gray matter, so the strain generally concentrates at the intersections of these two areas. In concussion, these strains are not associated with visible structural abnormalities on conventional neuroimaging. In a more severe traumatic brain injury, however, large magnitudes of force applied in the same fashion will frequently cause disruptions at the gray-white junction (i.e., diffuse axonal injury) (Fig. 29.4) [58].


Fig. 29.4
Characteristic hemorrhagic lesion in the corpus callosum in a patient with a fatal head injury from a motor vehicle accident. Note the paramedial distribution of the lesion affecting the full thickness of the corpus callosum. Adapted from Sahuquillo-Barris et al. Acute subdural hematoma and diffuse axonal injury after severe head trauma. J Neurosurg 68: 894–900, 1988
While human pathophysiology studies have tried to describe the motion of the brain in concussive injuries, the chemical and neuronal response to concussion has mostly been described in animal models [4, 14]. After the initial mechanical trauma to the brain, there is a complex neurochemical and neurometabolic response. Neuronal axons are stretched, leading to membrane deformation and a release of neurotransmitters and ions. This shift leads to an alteration in glucose metabolism and mitochondrial functioning. Cells respond by downregulating excitatory neurotransmitter receptors and eventually reset themselves to their normal state over several days. Depending on the severity of the injury, the mechanical stretching on axonal cell membranes can cause disruption of the cytoskeleton and likely reversible axonal injury.
Glutamate Release and Ionic Flux (Fig. 29.5)

Fig. 29.5
Summary of changes of all parameters in rats who recovered from trauma (Type I), suffered brain contusions (Type II), died (Type III), or who had only mild changes (Type IV), in which changes are demonstrated with mean value and standard deviation. Type I animals are believed to represent severe concussions, while Type IV represent more mild concussions. Adapted from Takahashi et al. Changes in extracellular plasma concentration in the cortex and brain stem during the acute phase of experimental closed head injury. J Neurosurg 55: 708–717, 1981
With the initial trauma, axonal stretching leads to membrane deformation and an indiscriminate flux of ions through previously regulated ion channels [22]. There is a large efflux of potassium and a release of excitatory amino acids, especially glutamate [37]. This release of glutamate leads to indiscriminate activation of neuronal cells through binding to NMDA and AMPA ionic channels [21]. At the same time, cells work to restore the normal ionic balance through the ATP-dependent Na+/K+ pumps. Due to the shift in ions beyond a normal physiologic level, cellular metabolism is strained and lactate production increases, leading to local acidosis, increased membrane permeability, and cerebral edema [36, 38, 104].
Alterations to Brain Metabolism and Activation
After an initial period of hyperglycolysis, lasting approximately 6 h, a prolonged period of glucose hypometabolism can occur [105]. Activation of NMDA channels leads to an influx of calcium ions, which accumulate in mitochondria and lead to dysfunction in glucose oxidation [43, 75, 100, 104]. In studies of humans with moderate to severe TBI , PET imaging has shown glucose hypometabolism to last for months [7]. While no short-duration, longitudinal, within-subject studies have confirmed it, it is believed that a similar disruption in mild TBI and concussion recovers much more rapidly.
Likely in response to over-activation by glutamate, NMDA receptors are downregulated by post-injury day 2–4 and appear to return to normal by day 7 [25]. NMDA channels are associated with learning and long-term potentiation (LTP ), and post-injury animal models have shown a decrease in LTP induction after post-injury day 2 [73, 84]. While this generally recovers after 1–2 weeks, it has been seen to take up to 8 weeks to fully return to normal [79]. Human fMRI studies have shown abnormal activation of neural circuits in patients with cognitive deficits at 1 week after injury [32]. When these changes have been observed, the affected individuals seem to have a prolonged clinical recovery [45, 50].
Axonal Injury
In addition to the neurometabolic effects that occur after axonal stretching, disruptions of the cytoskeleton can occur as well. Acutely, neurofilament compaction can occur due to phosphorylation or sidearm proteolysis [34, 92]. As calcium accumulates, microtubules can become destabilized [49]. Axonal transport can be interrupted, and eventually blebbing and disconnection can occur [68, 78].
This process has been observed in animal dissection studies and more recently in human studies using advanced MRI and DTI techniques (Fig. 29.6) [61, 88]. Using DTI , it has been observed that fractional anisotropy, a measure of linear water diffusion, is decreased in several white matter regions after mild TBI . These decreases have been correlated to decreased attention control and memory in adults, as well as to changes in motor speed, executive function, and behavior in children (Fig. 29.7) [62, 101].



Fig. 29.6
Region of interest (ROI) placement for DTI analysis. Shown are corresponding ROIs for the right hemisphere. The solid ellipse within yellow outline indicates the location and size of the ROI. (a) uncinate fasciculus, (b) inferior longitudinal fasciculus, (c) genu of the corpus callosum, (d) anterior corona radiata, (e) cingulum bundle, (f) superior longitudinal fasciculus. Adapted from Niogi SN et al., Structural dissociation of attentional control and memory in adults with and without mild traumatic brain injury, Brain, 2008, vol 131, issue 12, pp 3209–3221, by permission of Oxford University Press

Fig. 29.7
Two cognitive functions are specifically associated with white matter microstructure in two distinct regions in adults with mild TBI . (a) Correlation of memory performance and average bilateral uncinate fasciculus (UF) fractional anisotropy (FA) in both hemispheres (r = 0.52, P < 0.001). (b) The anterior corona radiata (ACR) FA does not correlate significantly with long delay free recall memory (r = −0.057, P = 0.725). (c) The UF FA does not correlate significantly with attentional control (r = 0.133, P = 0.41). (d) Correlation of attentional control measured by conflict score and the left ACR FA (r = 0.47, P – 0.001). Adapted from Niogi SN et al., Structural dissociation of attentional control and memory in adults with and without mild traumatic brain injury, Brain, 2008, vol 131, issue 12, pp 3209–3221, by permission of Oxford University Press
Acute Response to Repeat Concussion and the “Second Impact Syndrome”
The most dreaded consequence of concussion is the reported “second impact syndrome.” When a second concussion occurs in close temporal proximity to an initial concussion, a catastrophic syndrome of cerebral edema can result in coma, severe neurologic deficit, and death [10, 11, 80]. It is unclear how long concussion patients are at risk for second impact syndrome, leading to the extensive debate over return to play timelines [41, 71]. Several animal studies have demonstrated a period of transient metabolic and physiologic vulnerability that may exacerbate the cellular injury caused by repeated mild injury [44, 95, 97].
After the initial injury, the previously described alterations in metabolism and brain activity occur. In a rat model, repeated mild injuries occurring 3 days after an initial mild injury cause damage similar to that which occurs after a single severe TBI [97, 99]. When the second injury occurred after 5 days, the resulting perturbations were similar to the initial injury itself. Given that translating such a time window to humans is difficult, it is reasonable that individuals who have suffered from a concussion do not put themselves in harms way while they are still symptomatic from the initial injury.
Special Considerations in the Pediatric Population
While the majority of the biomechanical experiments on mild TBI have occurred in adult populations, there are a few special items to consider in the pediatric population. Given the relative size of the head to the rest of the body in children, angular motion of the head is likely intensified. In helmeted sports in particular, the added weight of the helmet itself compared to the weight of the head and body leads to an increase in angular motion and likely a proportional increase in the forces transferred to the brain [17]. While the overall smaller size of the brain in children may be somewhat protective (i.e., more force is required to cause injury), the increases in force described in helmeted sports likely negate this protection [65].
Evaluation of Concussion Patients
The most important part of the initial evaluation of a patient after a head impact is ruling out injury requiring surgical intervention. In the early 1990s, several studies reported that a substantial portion (up to 20 %) of patients with minor head injuries had intracranial lesions on CT based on retrospective data [28, 90, 91]. It was suggested based on this data that every patient with a minor head injury should have a CT scan. However, further prospective studies on patients with a GCS score of 15 showed an intracranial lesion rate of only 6–9 % on CT [33, 56, 57]. Studies have demonstrated the utility of clinical findings as predictors of intracranial lesions, with sensitivities of 96 and 98 % for intracranial lesions and 100 % sensitivity for patients requiring neurosurgical intervention [56, 106]. Two more recent studies have demonstrated even greater sensitivity (100 % experimental sensitivity), based on a number of clinical findings [29, 93]. The New Orleans Criteria for CT scan in patients with minor head injury as described by Haydel et al. were as follows:
- 1.
GCS score < 15
- 2.
Short-term memory deficit
- 3.
Intoxication
- 4.
Age >60 years
- 5.
Seizure
- 6.
Headache
- 7.
Vomiting
- 8.
Evidence of trauma above the clavicle
The second study by Stiell et al. led to the publication of the Canadian CT Head Rules:
High risk for neurological intervention
- 1.
GCS score < 15 at 2 h after injury
- 2.
Suspected open or depressed skull fracture
- 3.
Any sign of basal skull fracture (hemotympanum, “raccoon” eyes, cerebrospinal fluid otorrhea/rhinorrhea, Battle’s sign)
- 4.
Vomiting, two or more episodes
- 5.
Age 65 years or older
Medium risk for brain injury on CT
- 1.
Amnesia for events more than 30 min prior to impact
- 2.
Dangerous mechanism (pedestrian struck by motor vehicle, occupant ejected from motor vehicle, fall from height > 3 ft or five stairs)
Both of these criteria have been validated by multiple studies in their sensitivity for intracranial lesions and prediction of lesions likely to require surgical intervention [60, 77, 87, 89, 94]. These same validation studies have consistently found the Canadian CT Head Rule to be more specific and believe its implementation would lead to a decrease in the frequency of CT head scans for mild traumatic brain injury. Given the delicate balance between detecting lesions and avoiding unnecessary radiation of pediatric patients, these are important rules to consider during an initial evaluation.
Clinical History and Exam
When a patient is brought to you for an evaluation after concussion, it is important to perform a detailed history and physical. A history of headaches (especially migraines), prior concussion, depression, anxiety, post-traumatic stress disorder, and attention deficit hyperactivity disorder all increase the risk that a patient will endure prolonged symptoms. Female sex and a higher intelligence quotient are also associated with prolonged symptoms [55]. The patient’s pre-injury functioning should be assessed: How have they done in school? Do they have difficulties in any particular subject area? When did they meet important developmental milestones?
Regarding the concussion itself, it is important to note the patient’s initial response: Was there loss of consciousness? Does the patient remember the events leading up to the injury? Does the patient remember the events after the injury? Was there a convulsive event before or after? If the answer is “yes” to any of these questions, documenting the length of time (e.g., length of loss of consciousness, how many minutes were forgotten before or after) should also be done.
The next step generally involves a concussion symptom scale, such as the Pittsburgh Post-Concussion Scale (Fig. 29.8) [107]. In adults and older adolescents, you can ask the patient to fill out the scale before hand, rating each of 21 symptoms on a scale of 0–6, where 0 is no symptom and 6 is severe. In younger children, you may need to go through the symptoms individually to get a response. While younger children may have difficulty differentiating between a 3 and a 6 in terms of symptom severity, they generally understand if something is better or worse than previously (e.g., “is your headache worse today or was it worse yesterday?”). In our practice, we ask that patients fill out the scale for the evening of the injury, the day after the injury, and the day of the evaluation, which allows us to track symptom progress over time.


Fig. 29.8
The Pittsburgh Post-Concussion Symptom Scale . Adapted from MR Lovell and MW Collins, Neuropsychological assessment of the college football player, J Head Trauma Rehabil, Vol 13, issue 2, pp 9–26
Finally, a detailed neurological exam should be performed. In concussion, there should be no focal neurological deficits. However, it is not uncommon to see a decrease in short-term memory (e.g., recalling two of three objects 5 min after registration), coarse eye movements on visual pursuit, mild dysmetria, or gait/balance disturbances. Focal neurological deficits should be evaluated as neurological emergencies and triaged to the ER accordingly. If symptoms persist beyond 2–3 days, patients should be evaluated by a neurologist or another medical professional that specializes in concussions. Cognitive complaints (e.g., decreased attention, decreased memory) may require detailed testing by a neuropsychologist .
Sideline Testing
Several sideline assessment tools have been designed to aid in the evaluation of a concussion patient [51, 52]. While these tools have been widely studied, it is not recommended that these tools be used to decide if a patient can return to play after sustaining a head injury. If a concussion may have occurred and these tests are to be performed, then it is the current recommendation of multiple medical associations that the individual be withheld from same-day return to play and receive a complete evaluation from a fully licensed and trained medical professional. Several of the sideline assessment tools may be used as part of a clinical decision to allow someone to return to play, but their use as a stand-alone tool should be limited. When these tools are used, serial documentation of a subject’s performance should be documented, including baseline testing prior to participation in a sport. These tools allow for initial documentation of symptoms (e.g., headache), physical signs (e.g., LOC, amnesia), behavioral changes (e.g., irritability), and cognitive impairment (e.g., memory deficits) (Fig. 29.9) [53].


Fig. 29.9
Portion of the Child Sideline Assessment Tool 3 (Child SCAT3) . The full Child SCAT3 can be obtained free of charge from the website of the British Journal of Sports Medicine at bjsm.bmj.com/content/47/5/263.full.pdf. Reproduced from Br J Sports Med, McCrory et al., Vol 47, page 263, 2013 with permission from the BMJ Publishing Group Ltd
Neurocognitive Testing
Several neurocognitive testing paradigms have been developed for the evaluation of patients with concussion. The most widely used, the Immediate Post-Concussion Assessment and Cognitive Testing (ImPACT), scores patients with six modules and a symptom scale. It derives composite scores on verbal memory, visual memory, reaction time, visual motor processing speed, and impulse control to identify specific cognitive domains that a concussed patient may have difficulty with. In a recent study on high school athletes, researchers were able to correctly classify patients as control or post-concussion utilizing composite scores from the ImPACT test battery in approximately 85 % of cases [81].
Given the competitive nature of many athletes, relying on self-reported symptoms can lead to the underdiagnosis of concussion and premature return to play decisions. In one recent study, the sensitivity of concussion diagnosis increased by 19 % when self-reported symptom scores were combined with computerized neurocognitive testing , compared to symptom scores alone [98]. Further, neurocognitive testing can help determine if patients have fully returned to baseline after a concussion, even after previously reported symptoms have resolved [19, 24]. However, the limitations of neurocognitive testing alone have been demonstrated in a large study on professional football players, where evaluations by clinical staff kept athletes out of participation despite resolution of neurocognitive test scores due to other clinical variables [66].
As with sideline assessments, neurocognitive testing should not be interpreted in a vacuum. Testing can provide a window into specific areas that a subject may be deficient in and can help guide further diagnostic testing by neuropsychologists. It is most useful when patients have pre-participation testing performed to act as a baseline. However, in children, these tools are generally more limited given the varied pace at which individuals progress. Even baseline testing can be unreliable in children, as their scores on neurocognitive tests can improve dramatically over time, and peer-to-peer comparisons are frequently just as fraught with variation.
More formalized neuropsychological testing, performed by a psychologist specially trained in cognitive testing, may be indicated if patients exhibit chronic deficits in any cognitive domains, even if their overall functioning has appeared to return to baseline. It is important that children especially get these evaluations to insure their return to school occurs in an organized and productive manner.
Neuroimaging
As described at the beginning of this section, the vast majority of patients who suffer concussions do not require neuroimaging. Standard CT and MRI imaging will be negative in a concussion, but can be used to evaluate for more serious brain injuries. CT is useful for the evaluation of bone fractures, intracranial bleeding, cerebral contusions, and general mass effects; it should only be used in the acute setting if clinically indicated and is generally not useful in patients with persistent symptoms. MRI , on the other hand, is more sensitive for evaluating symptoms that worsen or persist beyond a reasonable period of time. For example, if a patient has headaches that start to worsen or become positional, if they develop seizures after the injury, or if they have persistent balance or neurocognitive deficits, MRI can be used to evaluate brain architecture very finely.
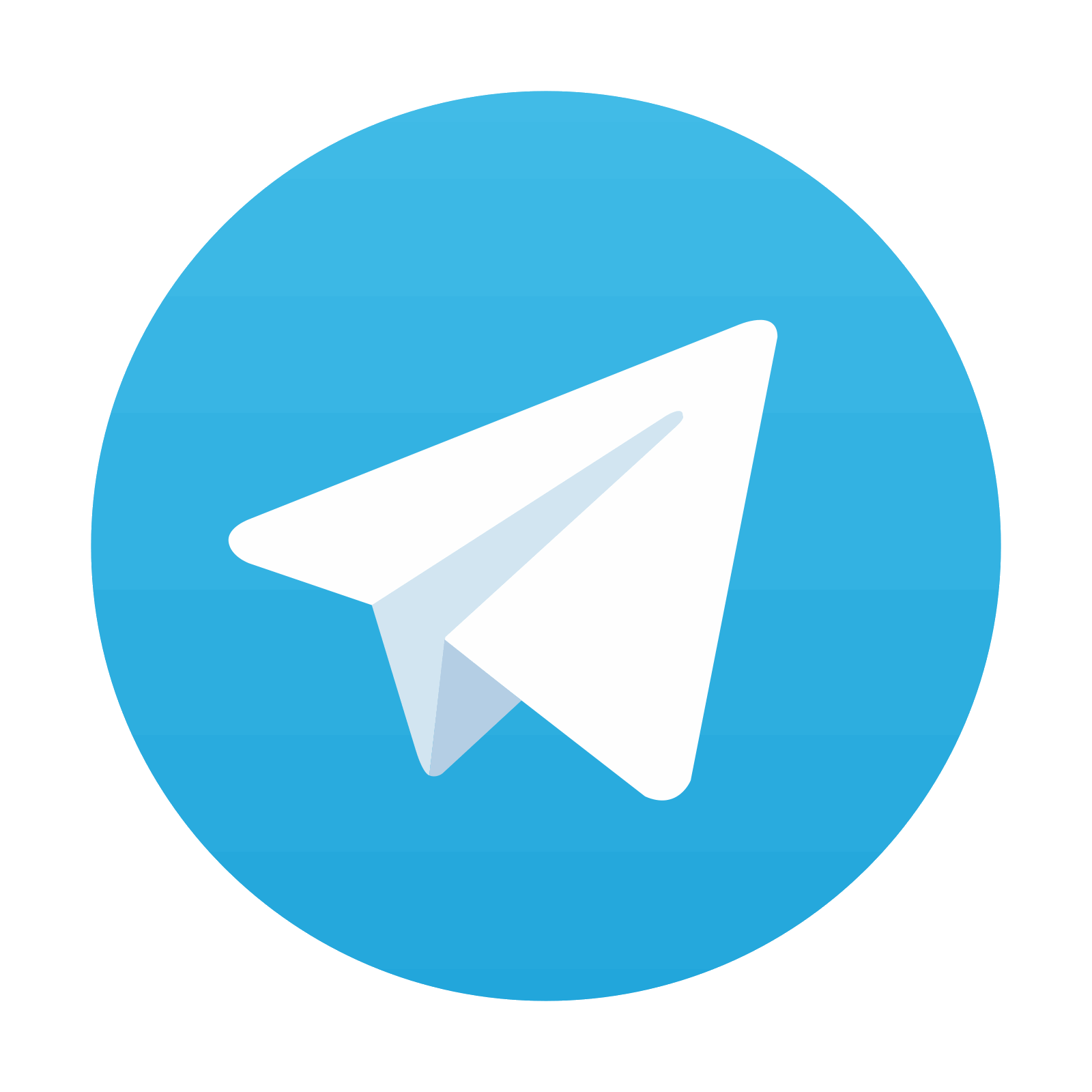
Stay updated, free articles. Join our Telegram channel
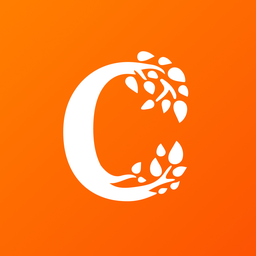
Full access? Get Clinical Tree
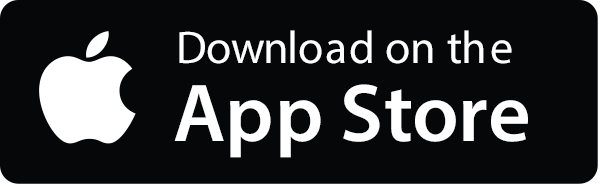
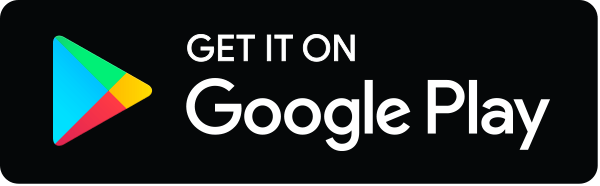