Abstract
Hypoxic-ischemic encephalopathy in the perinatal period is a common disorder that has an impact throughout the perinatal period in both the preterm and term-born infant. Hypoxic-ischemic encephalopathy is characterized by neuropathological and clinical features that constitute an important portion of neonatal neurology. To understand those features, which are discussed extensively in this Unit IV, it is necessary to be cognizant of the pathophysiological underpinnings, including the biochemical and physiological derangements, that lead to the structural and functional manifestations of this encephalopathy. Hypoxia refers to deficiency in oxygen within the circulation and at the cellular level. Ischemia refers to insufficient perfusion or, more specific to this setting, cerebral blood flow. This will usually be associated with concurrent hypoxia at the cellular level. The term hypoxic-ischemic injury is often used because of the intimate nature of these two components in mediating cerebral injury in the newborn infant. Thus, key to all is hypoxia and energy deprivation (including glucose) at the cellular level, resulting in alterations in cellular metabolism, with interruption of typical cellular function and a series of aberrant cellular consequences. In this chapter, we first deal with the major modes of cell death in the setting of hypoxic-ischemic injury. We then review the fundamental derangements in energy and cerebral blood flow, on a background of the normal cerebral biochemistry and circulation, of the perinatal brain. Much of what we know is based on experimental data, but translational data in humans with neuroimaging support these concepts. The secondary effects of these derangements in energy and cerebral blood flow via excitatory, oxidative, and inflammatory pathways is discussed. This will form the foundation for reviewing approaches to neuroprotection in the term and premature brain in relation to hypoxic-ischemic injury. The applications of these principles and their related neuropathologies in the setting of the preterm and term infant’s brain is discussed in more detail in the later chapters within this unit.
Keywords
Hypoxic-ischemic brain injury, energy failure in the newborn brain, cellular processes, apoptosis, necrosis
Hypoxic-ischemic encephalopathy in the perinatal period is characterized by neuropathological and clinical features that constitute an important portion of neonatal neurology. To understand these features, which are discussed extensively in this unit, it is necessary to be cognizant of the pathophysiological underpinnings, including the physiological and biochemical derangements, that lead to the structural and functional manifestations of this encephalopathy. In this chapter, we first deal with the major modes of cell death in the setting of hypoxic-ischemic injury. We then review the fundamental derangements in cerebral blood flow (CBF) and energy metabolism, on a background of the normal cerebral circulation and biochemistry of the perinatal brain. Much of what we know is based on experimental data, but translational data in humans with neuroimaging supports these concepts (see later). The subsequent effects of these derangements in CBF and energy metabolism, via excitatory, oxidative, and inflammatory pathways, will then be discussed. These insights will form the foundation for reviewing approaches to neuroprotection in the term and premature brain in relation to hypoxic-ischemic injury. The applications of these principles and their related neuropathologies in the setting of the preterm and term infant’s brain will be discussed in more detail in the later chapters within this unit.
Definitions
It seems worthy at the commencement of this chapter and unit to review the key definitions that will be used. Hypoxemia refers to deficiency in oxygen within the circulation and at the cellular level. Ischemia refers to insufficient perfusion or, more specific to this setting, insufficient CBF. This deficit will usually be associated with concurrent hypoxia at the cellular level. The term hypoxic-ischemic injury is often used, due to the intimate nature of these two components in mediating cerebral injury in the newborn infant. Finally, asphyxia refers to an impairment of respiratory gas exchange and therefore a concomitant increased pCO 2 and acidosis combined with hypoxia. Asphyxia is usually associated with alterations in CBF, with the increased pCO 2 initially having enhanced CBF via vasodilatory effects. Subsequent impairment of CBF is then usual. Thus central to all mechanisms (hypoxemia, ischemia, and/or asphyxia) is hypoxia and subsequent substrate deprivation .
Finally, the terms neonatal encephalopathy and hypoxic-ischemic encephalopathy should be clarified. Neonatal encephalopathy refers to altered behavior in the newborn characteristic of a disturbance in central nervous system functioning. The major etiology of neonatal encephalopathy is hypoxic-ischemic injury, and once identified as the etiology the infant’s condition can be more specifically referred to as hypoxic-ischemic encephalopathy, or an encephalopathy resulting from a hypoxic-ischemic insult.
Mode of Cell Death—Necrosis, Apoptosis, and Autophagy
Before defining the underlying biochemical and physiological principles that determine cell death in the immature central nervous system, it is important to delineate the major modes of cell death and key factors leading to cell death. Two fundamental modes of cell death in the nervous system, as in other tissues, are distinguished: necrosis and apoptosis ( Fig. 13.1 ). It is now clear that hypoxic-ischemic insults may lead to necrosis or apoptosis, or more commonly a continuum, dependent principally on the severity of the insult and the maturational state of the cell. Certain characteristics readily distinguish these two forms of cell death ( Table 13.1 ). Thus necrotic cell death is characterized by cell swelling, membrane disintegration, cell rupture, release of intracellular contents, and, as a consequence, inflammation and phagocytosis. By contrast, apoptosis is characterized by condensation and margination of chromatin, cell shrinkage, relative preservation of cellular membranes, and death without inflammation or alternative.

DISTINGUISHING FEATURE | NECROSIS | APOPTOSIS |
---|---|---|
Morphology | Cell swelling; dispersed chromatin; membrane fragmentation; inflammatory responses | Cell shrinkage; chromatin condensation; intact membranes; no inflammation |
DNA fragmentation | Nonspecific | Specific oligonucleosomal cleavage |
Involvement of specific death genes/enzymes (e.g., Bax, Bid, p53, AIF, PARP, cytochrome c, caspases) | No | Yes |
Adenosine triphosphate required | No | Yes |
Protein synthesis required | No | Yes |
Temporal characteristics | Usually rapid (minutes to hours) | Slow (hours to days) |
Insult characteristics | More severe | Less severe |
Apoptotic cell death is difficult to detect in tissue because of the lack of inflammation and the rapid removal of the cell debris. Apoptotic cell death requires activation of specific death genes, adenosine triphosphate (ATP), and new protein synthesis, which result particularly in a series of biochemical changes that include cleavage of DNA at specific sites to result in the characteristic oligonucleosomal fragmentation (see later). Necrotic cell death occurs typically after intense, sometimes relatively brief insults, whereas apoptotic cell death occurs typically after less intense, longer acting insults. Apoptotic cell death appears to be the dominant form of so-called delayed cell death, observable after many hours to several days in various experimental neonatal models and human brain. Important intrinsic properties of the cell itself determine the mode of cell death relating particularly to the developmental stage of the cell. Thus the susceptibility to apoptosis is enhanced in immature versus mature neurons in vitro and in vivo. Apoptotic cell death was noted to be common in a study of infants who died after intrauterine hypoxic-ischemic insult (see Chapter 18 ). Moreover, careful studies in the neonatal piglet subjected to hypoxia-ischemia demonstrated in the same paradigm exclusively necrotic cell death in certain neuronal populations, both necrosis and apoptosis in other neuronal populations, but exclusively apoptotic cell death in immature cerebral white matter. Indeed, in many models, electron microscopic study reveals an apoptotic-necrotic continuum in neuronal regions, with clearly apoptotic and necrotic cells present, as well as hybrid cells with “intermediate” characteristics. Often the early cell death appears necrotic and later cell death apoptotic ( Fig. 13.2 ).

The molecular mechanisms involved in apoptosis associated with neonatal hypoxia-ischemia have been clarified considerably, although not completely, in recent years. Although many molecular triggers of apoptosis exist, perhaps most important with hypoxia-ischemia, influx of Ca 2+ and generation of ROS (see later) are membrane perturbations that involve release of ceramide from sphingomyelin, translocation to the mitochondrion of proapoptotic members of the Bc1-2 family (i.e., Bax, Bid), formation of a mitochondrial permeability pore, release from mitochondrion of cytochrome c and apoptosis-inducing factor, and stimulation of endonucleases by apoptosis-inducing factor and of caspases, especially caspase-3 by cytochrome c. Both caspase-dependent and caspase-independent mechanisms of apoptotic cell death have been recognized . Caspases are proteases with cysteine residues in their active sites and catalyze proteolysis at specific aspartate residues—hence the term caspase . Caspase-3 is activated within 1 to 3 hours after neonatal hypoxia-ischemia and is a principal executioner of apoptosis. The substrates attacked by caspases include cytoskeletal and associated proteins, nuclear and DNA-associated proteins, signal transduction proteins, ion channel subunits, and other key molecules. One of the results of the process is DNA cleavage, and poly(ADP ribose) polymerase (PARP), involved in DNA repair, is activated. PARP activation is a prominent feature of the caspase-dependent apoptotic cascade. PARP inactivation occurs by caspase-3–mediated cleavage during the early stages of apoptosis. This inactivation facilitates nuclear disassembly and ensures the completion of the apoptotic process, because PARP activation depletes the cell of ATP, which is essential for apoptosis. The action of apoptosis-inducing factor to cause apoptotic cell death is caspase- independent and involves principally translocation to the nucleus and activation of endonucleases. The complex molecular cascades involved in apoptosis occur over many hours and days, thus raising the possibility of interruption of the cascade during a relatively long time window (see later).
More recent attention has been paid to forms of neurodegeneration in the developing brain, which have been termed “pathological apoptosis” and excitotoxic neurodegeneration. Due to the fact that this process rests on a continuum between necrosis and apoptosis, it has also been titled “necroptosis.” The important contribution of these regulated but morphologically hybrid forms of cell death to hypoxic-ischemic injury in the newborn brain is emerging.
These regulated forms of cell death are good examples of molecular switching between apoptotic and necrotic modes of cell death. An additional regulated form of cell death, programmed necrosis, is increasingly recognized as a key form of neurodegeneration and also lies along the apoptosis necrosis continuum. The contribution of these neurodegenerative forms of neuronal cell death in the neonatal brain are still under active investigation.
A final form of cell loss, autophagy, also occurs within the setting of neonatal hypoxic ischemic brain injury. Autophagy is an adaptive process through which eukaryotic cells degrade and recycle their own cytoplasm and organelles via lysosomes, in response to unfavorable conditions. Autophagy is considered to be a homeostatic nonlethal stress response protecting the cell from low nutrient supplies. Autophagy is classified as a form of programmed cell death. A histological hallmark of autophagy is the formation of double-membrane autophagosomes derived from the endoplasmic reticulum. These then mature and fuse with lysosomes, followed by degradation or recycling of the autophagosome content. Autophagy is seen in developmental and pathological conditions, and both in vitro and in vivo studies reveal that it has a significant role after neonatal hypoxic-ischemic injury, depending on the severity of the insult, maturation, and cerebral region.
Importance of the Reperfusion Period
As discussed in the next section, the cascade of deleterious events that lead to cell death after insults that result in oxygen deprivation and energy failure appears to occur primarily following the termination of the insult . Careful studies in animal models and in human patients provide strong support for this notion. The phenomena are initiated particularly by energy depletion, accumulation of extracellular excitatory amino acids (particularly glutamate), increase in cytosolic Ca 2+ , and generation of free radicals. The importance of this “delayed” death of brain in the hours after termination of the insult is related in the largest part to the possibility that intervention during the postinsult period may be beneficial. Data to support this possibility are now available, with the effectiveness of postnatal neuroprotective approaches, such as therapeutic hypothermia, discussed later.
Initiating Role of Energy Failure
An understanding of the mechanism of cell death in the setting of hypoxic-ischemic injury requires appreciation of the cascade of interrelated cellular events. We will commence with the principal underpinnings of cell death related to energy depletion, which is most commonly the result of ischemic injury resulting from a failure of adequate CBF . In earlier years, cell death with oxygen deprivation was explained entirely by reference to the sharply decreased production of high-energy phosphates from anaerobic glycolysis ( Figs. 13.3–13.5 ). The mechanism cited was deficiency of high-energy phosphates that are necessary for synthesis of macromolecules and lipids, and thus maintenance of structural integrity. Several decades of research have made it clear that this explanation is too simple and that cell death does not require energy depletion severe enough to eliminate the synthesis of structural components. However, an initial decrease in high-energy phosphates is capable of triggering a series of additional mechanisms that begin with a failure of the ATP-dependent Na + -K + pump. If the insult is very severe, the acute result is Na + influx, followed by chloride (Cl − ) and water influx, cell swelling, cell lysis, and thus early cell death by necrosis ( Fig. 13.6 ; see also Fig. 13.1 ).




In the more typical, less severe insult, membrane depolarization occurs and is followed by extracellular accumulation of glutamate, increased cytosolic Ca 2+ , and a cascade of events leading to a more delayed cell death , principally apoptotic , although necrosis may also occur by this mechanism. The details are discussed in the ensuing sections. Suffice it to say here that the increased extracellular glutamate results from (1) excessive presynaptic glutamate release (because of membrane depolarization and later increased cytosolic Ca 2+ ) and (2) failure of glutamate uptake mechanisms in presynaptic nerve endings and astrocytes (because of membrane depolarization and failure of high-affinity, Na + -dependent glutamate transporters). The increase in cytosolic Ca 2+ is a consequence of (1) failure of energy-dependent Ca 2+ -pumping mechanisms, (2) opening of voltage-dependent Ca 2+ channels (secondary to membrane depolarization), and probably most important, (3) activation of specific glutamate receptors (see later discussion). The subsequent deleterious events provoked by Ca 2+ and leading to cell death after these initial events are described later. The pathway to cell death with hypoxic-ischemic insults begins with derangements in CBF, and thus in the following we first discuss CBF and its regulation, and then the biochemical effects of disturbed CBF.
Primary Mediators of Hypoxic-Ischemic Injury in the Newborn Brain—Physiological and Biochemical
Major Pathogenetic Themes
The traditional unifying disturbance to neural tissue in hypoxic-ischemic encephalopathy is a deficit in oxygen supply resulting in energy deficit. The perinatal brain can be deprived of oxygen by two major pathogenetic mechanisms: hypoxemia , which is a diminished amount of oxygen in the blood supply, and ischemia , which is a diminished amount of blood perfusing the brain. The balance of experimental and clinical data leads to the conclusion that ischemia is the more important of these two forms of oxygen deprivation . Thus the initial focus of this chapter will be CBF and its perturbation. The conclusion that ischemia is of pivotal importance suggests that more than deprivation of oxygen is required, supporting that deprivation of glucose as well as oxygen is crucial in the genesis of injury. Moreover, the period of reperfusion now has been shown clearly to be the time of occurrence of many, if not most, of the deleterious consequences of ischemia on brain metabolism and, ultimately, structure (see later discussion). In most instances, during the perinatal period, hypoxemia or ischemia or both occur as a result of asphyxia , which refers to impairment in the exchange of respiratory gases, oxygen, and carbon dioxide. Thus, in asphyxia, the major additional feature is hypercapnia, which results in other metabolic (e.g., additional acidosis) and physiological (e.g., initial increase in CBF) effects. In the following sections, we first discuss CBF in the immature brain and its patterns of perturbation in the preterm and term-born brain. In the next major section, we then discuss the biochemical changes in the brain associated with hypoxemia, ischemia, and asphyxia, initially with an emphasis on carbohydrate and energy metabolism. The manner in which these biochemical changes are affected by other perinatal factors (e.g., the status of carbohydrate metabolism at the time of the insult, the state of brain maturation, and the process of birth) is also described. Subsequent sections synthesize the burgeoning literature on the mechanisms of cell death with oxygen deprivation and focus on the critical importance of biochemical events beyond glucose and energy metabolism. Particular roles for increase in extracellular glutamate, excessive activation of glutamate receptors (excitotoxicity), increase in cytosolic calcium (Ca 2+ ), and generation of free radicals are emphasized.
Physiological Mediators: Cerebral Blood Flow
Importance of Cerebral Blood Flow and Regulation
Extensive clinical and neuropathological data emphasize the major role of ischemia in the genesis of brain injury associated with adverse perinatal events. Thus alterations in CBF are of prime importance for understanding the neuropathological and neurological consequences of all varieties of perinatal asphyxial and hypoxic-ischemic insults, as well as the pathogenesis, prevention, and treatment of these consequences. In the following discussion, we review CBF, its regulation, and the changes associated with asphyxia and related hypoxic-ischemic insults. By necessity, the discussion involves studies with experimental animals. However, growing experience with the human newborn, described in the final sections, indicates that the lessons learned from animal research are largely relevant to the perinatal human.
Cerebral Blood Flow: Knowledge From Experimental Studies in Animal Models
Fetal Circulation
The essential features of the fetal circulation, based principally on work with large animals (e.g., sheep, goats, and nonhuman primates), begin with events at the placenta. Gas exchange occurs efficiently at the placenta, although oxygen diffusion is somewhat restricted, and fetal arterial oxygen tension values are considerably lower than maternal values. Compensatory responses to this lower oxygen tension in the fetus include hemoglobin F, with its favorable oxygen affinity curve, polycythemia, and a relatively high cardiac output. Oxygenated blood from the placenta is carried through the umbilical vein, which empties into the inferior vena cava. This well-oxygenated blood enters the right atrium and is preferentially shunted through the patent foramen ovale, ultimately to the aortic arch and then to the coronary and cerebral circulations. Poorly oxygenated blood from the superior vena cava is preferentially shunted into the right ventricle and the pulmonary artery. Because of the high pulmonary vascular resistance, this blood primarily enters the ductus arteriosus and the descending aorta and returns to the placenta through the umbilical arteries.
Regulation of Cerebral Blood Flow: General Principles
CBF in experimental animals has been measured principally by techniques based on the clearance of an inert gas (e.g., xenon and nitrous oxide), carotid artery flow determinations, [ 14 C]antipyrine infusion with autoradiography, and infusion of radioactive microspheres with subsequent tissue sampling. In recent years, near-infrared spectroscopy, sometimes combined with infusion of indocyanine green, and magnetic resonance imaging techniques have been used. Because considerable variability in absolute values of CBF is observed with different techniques, species, modes of anesthesia, preparation of animals, and so forth, we have placed most emphasis in this discussion on the major conclusions of the many studies rather than on the absolute values of CBF recorded. The focus of this section is on the general principles of cerebral hemodynamics, primarily in mature animals; immature animals are discussed subsequently in a separate section.
Autoregulation.
Autoregulation of CBF refers to the maintenance of a constant CBF over a broad range of perfusion pressures. This constancy of CBF results from arteriolar vasoconstriction with increased perfusion pressure and vasodilation with decreased perfusion pressure. The mechanisms underlying autoregulation are not entirely understood. Currently the balance of data suggests that autoregulation is mediated primarily by an interplay between endothelial-derived constricting and relaxing factors (see the later discussion of perinatal CBF). Autoregulation in the adult human is operative over a range of mean blood pressure between approximately 60 and 150 mm Hg, and the response time is approximately 3 to 15 seconds.
Coupling of Cerebral Function, Metabolism, and Blood Flow.
Tight coupling of cerebral function, metabolism, and blood flow is well established and can be demonstrated by a variety of correlative physiological, biochemical, and even clinical studies. This coupling appears to be mediated by regulation of CBF by one or more local chemical factors that are vasoactive. Vasoactive factors of importance in the brain include H + , K + , adenosine, prostaglandins, osmolarity, and Ca 2+ ( Table 13.2 ). Increase in the perivascular H + concentration (i.e., decrease in local pH) is associated with arteriolar vasodilation. Greater neuronal metabolic activity can decrease local pH and therefore increase substrate supply. The effect of perivascular H + concentration mediates the vasodilating action of arteriolar carbon dioxide and is important under a variety of other physiological and pathological conditions (see later discussion). This vascular response is well established in the perinatal brain (see later section). K + has a vasoactive effect. Vasodilation increases linearly with extracellular K + levels to 10 mmol/kg (levels >20 mmol/kg induce vasoconstriction). The vasodilation is mediated by a Ca 2+ -activated K + channel on vascular smooth muscle. Because K + is released from nerve cells with electrical activity or a variety of insults, including oxygen deprivation (see earlier discussion), this ion may play a role in the regulation of CBF under certain pathological conditions.
TISSUE | PHOSPHOCREATINE | ADENOSINE TRIPHOSPHATE | GLUCOSE | LACTATE |
---|---|---|---|---|
Control | ||||
Parietal cortex | 2.74 ± 0.08 | 2.30 ± 0.08 | 2.38 ± 0.25 | 1.08 ± 0.09 |
Subcortical white matter | 1.85 ± 0.22 | 1.64 ± 0.06 | 2.14 ± 0.13 | 1.34 ± 0.07 |
Hypoxia | ||||
Parietal cortex | 2.56 ± 0.06 | 2.26 ± 0.02 | 1.64 ± 0.28 | 12.0 ± 1.4 |
Subcortical white matter | 1.09 ± 0.19 | 1.40 ± 0.09 | 0.28 ± 0.04 | 13.4 ± 1.8 |
Adenosine , administered on the perivascular side of pial arteries, results in a concentration-dependent vasodilation. Changes in adenosine also accompany certain pathological states with changes in CBF, including oxygen deprivation. Prostaglandins , particularly prostaglandins E and F 2 , lead to cerebral vasodilation. The concentrations of these compounds increase in response to cerebral ischemia, and agents that inhibit prostaglandin biosynthesis (e.g., indomethacin) have cerebral vasoconstrictive effects. Prostaglandins appear to be of cerebral hemodynamic importance in immature animals (see later discussion). Increases in perivascular osmolarity have a vasodilating effect, and decreases have a vasoconstricting effect. These data may bear on such effects on CBF as the vasodilation associated with infusion of hypertonic solutions. Ca 2+ may also play a role in the control of CBF (e.g., high perivascular concentrations of Ca 2+ lead to vasoconstriction, and low concentrations lead to dilation of cerebral vessels). The ability of Ca 2+ channel blockers to lead to increases in CBF relates to the prevention of the vasoconstricting effects of Ca 2+ . Extracellular Ca 2+ concentrations decline with hypoxia (see earlier discussion) and status epilepticus. Other chemical factors (e.g., renin-angiotensin, vasopressin, endogenous opioids, other neuropeptides, adrenergic compounds, acetylcholine, and endothelium-derived relaxing and constricting factors) may play important roles in the regulation of CBF, but more data are needed on these issues. Limited data are available on these agents in immature animals. One report shows that morphine infusions in neonatal piglets result in an upregulation of the vasoconstrictor, endothelin-1, and its receptors.
Cerebral Blood Flow in the Perinatal Period
Ontogenetic Effects.
Total and regional CBF changes significantly with maturation. In general, CBF overall increases with postnatal age, and this increase correlates well with similar increases in cerebral metabolic rates and energy demands, and with neuroanatomical development. Changes in regional CBF with maturation also reflect coupling with metabolic and anatomical development . The most dramatic short-term ontogenetic change in CBF occurs around the time of birth. In the lamb, CBF decreases by approximately threefold in the first 24 hours after birth. This decrease correlates well with the postnatal increase in oxygen content ( Fig. 13.7 ), consistent with the importance of oxygen delivery in the regulation of CBF.

Regional Effects.
Impressive regional differences in CBF are apparent in the perinatal animal, and these differences relate in considerable part to regional differences in metabolic activity. Using the microsphere technique to study regional CBF in term fetal sheep, Ashwal et al. initially noted (1) higher flows in brain stem than in cerebrum and (2) higher values in cortical gray matter than in subcortical white matter. Cavazutti and Duffy amplified these findings in a study of blood flow to 32 brain regions in the newborn dog, confirming the highest CBF in cerebral gray matter, nuclear structures of the brain stem, and diencephalon and lowest in cerebral white matter. Blood flows to the cerebral cortex were approximately 5- to 10-fold of those to subcortical white matter. These regional differences have been confirmed in studies of the perinatal rabbit, lamb, piglet, and puppy. Parallel studies of regional CBF and cerebral glucose metabolism ([2- 14 C]deoxyglucose method) demonstrated a close correlation with CBF, thus indicating that the coupling of blood flow and metabolism is present in the neonatal and adult animal. Finally, studies of blood flow to various regions of primate cerebrum indicate that the parasagittal regions , especially in the posterior aspects of the cerebral hemispheres, have significantly lower flow than other cerebral regions. This finding may have major implications for the distribution of brain injury with perinatal ischemic insults (see the section on parasagittal cerebral injury in Chapter 20 ).
Cerebral Autoregulation.
Cerebral autoregulation appears to be operative over a broad range of arterial blood pressure in the preterm and term fetal lamb, the neonatal lamb, and the neonatal dog. The principal stimulus for the autoregulatory change in vascular diameter appears to be induced largely by the deformation of endothelial cells and generation of endothelial-derived signals that act on the vascular smooth muscle. With a decrease in transmural pressure, NO- and Ca 2+ -activated K + channels are important in the vasodilation response, and with an increase in transmural pressure, endothelin-1 is critical in mediation of the vasoconstriction response. The autoregulatory range of blood pressures varies slightly among species and experimental conditions. The curve for the preterm lamb at approximately 80% gestation is shown in Fig. 13.8 . The curve for the preterm lamb differs from that for the term or neonatal lamb in two respects. First, the autoregulatory range in the preterm lamb is narrower , especially at the upper limit of the curve. Second, and perhaps more strikingly, the normal arterial blood pressure in the preterm lamb is very near or at the lower autoregulatory limit . Indeed, in the preterm lamb at 80% of gestation, normal arterial blood pressure is only 5 to 10 mm Hg above the lower limit of the curve, in contrast to the situation in older animals. In a subsequent study that included preterm fetal lambs at approximately 65% gestation (i.e., the onset of the third trimester), the lower autoregulatory limit was essentially identical to the normal resting arterial blood pressure. More recent data indicate that the range of blood pressure over which autoregulation is operative decreases with lower gestational age. These data indicate that with decreasing gestational age, resting mean arterial blood pressure (MABP) values approach the lower limit of the autoregulatory plateau, and the range of blood pressure over which CBF remains constant narrows . Stated in another way, the observations suggest that the margin of safety, at least in the preterm fetus, and to a lesser extent in the term fetus, is small at the lower end of the autoregulatory curve and points to vulnerability to ischemic brain injury with modest hypotension , particularly in the preterm animal. Vulnerability to hypertension also may result because little change occurs in the upper limit of the autoregulatory range during a brief developmental period (third trimester in the lamb and the human) when normal arterial blood pressure increases markedly. Thus normal arterial blood pressure shifts precariously close to the upper autoregulatory limit and renders capillary beds (e.g., germinal matrix) vulnerable to hemorrhage with modest hypertension.

Autoregulation in the term fetal lamb and in the newborn lamb has been shown to be sensitive to hypoxia . Changes in PaO 2 from 20 to 16 mm Hg in the fetal animal and from approximately 70 to 30 mm Hg in the newborn animal abolished autoregulation. These decreases in PaO 2 resulted in decreases in arterial oxygen saturation of less than 50%, which can be considered a hypoxic threshold for impairment of cerebrovascular autoregulation. The impairment of autoregulation required only a 20-minute exposure to hypoxia, and autoregulation did not recover until 7 hours after restoration of normoxia . Studies in adult animals showed that autoregulation is abolished in the presence of hypercarbia , and a similar phenomenon was observed in the perinatal animal and in the human preterm newborn. In a single study of the newborn lamb, systemic acidosis also was shown to cause a loss in cerebrovascular autoregulation .
Regional variation in the decrease in CBF provoked by hypotension to blood pressure values below the lower limit of the autoregulatory plateau has been described in the neonatal piglet, puppy, and lamb. In the neonatal piglet, the percentage of reduction in blood flow was least to the brain stem and greatest to the cerebrum. In a more detailed regional study in the newborn puppy, the flow to cerebral white matter was most vulnerable to hypotension . Similarly, in the preterm lamb, the lower autoregulatory limit with hypotension was lower in the brain stem than in the cerebrum. Perhaps even more important, in the preterm lamb at the start of the third trimester, blood flow to cerebral white matter not only was particularly vulnerable to hypotension but also did not recover under conditions of reperfusion that restored blood flow to all other brain regions ( Figs. 13.9 and 13.10 ). The latter observations may have implications for the topography of the injury in the immature human brain with hypoxic-ischemic insults (see the following discussion and Chapter 14 , Chapter 15 , Chapter 16 ).


Changes in arterial PCO 2 (i.e., PaCO 2 ) have marked effects on CBF in perinatal as in adult animals. In a study of blood flow to 32 brain regions of the newborn dog, a positive linear correlation was obtained in each structure examined. However, the response to carbon dioxide varied widely among brain regions, ranging from an increase of only 0.15 mL/100 g per minute per mm Hg in PCO 2 in subcortical white matter to an increase of 4.8 in the vestibular and superior olivary nuclei. The limited vasodilatory response in cerebral white matter may have implications for the vulnerability of this region to hypoxic-ischemic injury. In general, the higher the blood flow to a particular structure, the greater the vasodilatory response to increasing PCO 2 ( Fig. 13.11 ).

The effects of profound hypocarbia on CBF are also of importance , as it occurs frequently in sick infants requiring substantial respiratory support. Studies in neonatal lambs indicated an abrupt decrease in CBF with hypocarbia induced by hyperventilation. The decrease was nonlinear, such that the vasoconstricting effect of hypocarbia declined at lower PaCO 2 tensions. Moreover, the decline in CBF became less prominent with time, such that CBF was no longer statistically different in severely hypocarbic animals (PaCO 2 = 15 mm Hg) compared with control animals (PaCO 2 = 36 mm Hg) after 6 hours ( Table 13.3 ). Perhaps most important, the declines in CBF did not cause any change in cerebral metabolic rate for oxygen because cerebral oxygen extraction fraction increased. The attenuation of the decline in CBF with increasing duration of hypocarbia probably relates to an increase in perivascular H + concentration, primarily secondary to an increase in lactate levels. This formulation is supported by the marked increase in CBF above baseline levels on restoration of normocarbia.
↑ Glucose influx to brain |
Link to accelerated glucose utilization |
↑ Glycogenolysis |
Phosphorylase activation (↑ cAMP) |
↑ Glycolysis |
Phosphofructokinase activation (↑ cAMP, ↑ ADP, ↑ P i , ↓ ATP, ↓ phosphocreatine) |
Hexokinase activation (↑ cAMP) |
↓ Brain glucose |
Glucose utilization > glucose influx |
↑ Lactate (and hydrogen ion) |
Anaerobic glycolysis |
Impaired utilization of pyruvate (through mitochondrial citric acid cycle–electron transport system) |
↓ Phosphocreatine |
↑ Hydrogen ion production through anaerobic glycolysis |
↓ ATP, ↑ ADP |
↓ ATP |
↓ Oxidative phosphorylation |
The effects of modest hypocarbia (e.g., PaCO 2 = 26 mm Hg) interact adversely with hypoxic-ischemic insults in the 7-day-old animal. Thus animals exposed to hypoxia-ischemia sustained a greater decline in CBF and a greater degree of brain injury when they were simultaneously rendered modestly hypocarbic, compared with animals who were normocarbic (PaCO 2 = 39 mm Hg) or rendered mildly hypercarbic (PaCO 2 = 54 mm Hg). The latter two groups had better preserved CBF, less severe cerebral metabolic deficits, and less severe brain injury. The modestly hypercarbic animals had the most favorable hemodynamic, biochemical, and neuropathological outcomes. However, more marked hypercarbia accentuated brain injury, because of marked decrease in CBF. The latter effect is presumably related to impaired autoregulation and cardiovascular depression.
Alterations in arterial PO 2 (PaO 2 ) also cause distinct changes in CBF. a
a References .
Decreases in oxygen tension result in increases in blood flow and vice versa. Jones and coworkers demonstrated that cerebral oxygen delivery is maintained by this increase in CBF over a wide range of arterial oxygen content. In a study of 32 brain regions of the newborn dog, as with PaCO 2 , the magnitude of the vasodilatory response to hypoxia varied. Hypoxia caused the largest percentage increases in regional blood flow in brain stem structures, moderate increases in cortical and diencephalic structures, and smallest increases in cerebral white matter. These observations again suggest that cerebral white matter has limited vasodilatory capacity and thereby has implications for the vulnerability of this region to hypoxic-ischemic injury in the preterm infant (see later discussion and Chapter 15 ). The mechanisms of the increase in CBF with hypoxia are likely to be related to local metabolic factors and to vascular factors per se. Thus, with hypoxia, rapid local increases in vasodilatory metabolic factors (e.g., perivascular H + , K + , adenosine, and prostaglandins) and decreases in vasoconstricting factors (e.g., Ca 2+ ) occur. In addition, strikingly rapid decreases in isometric tension generated by the major cerebral arteries isolated from near-term fetal lambs and studied in vitro as isolated segments have been shown with PO 2 lowered to 15 mm Hg. The relaxation was much faster than that observed in adult cerebral arterial segments. Whether this effect is related to local release of NO or another factor remains to be determined. However, the findings indicate that the myogenic properties of the major cerebral vessels themselves must be considered in the evaluation of mechanisms of changes in cerebral hemodynamics. Importance for the large cerebral vessels in regulation of CBF in the preterm human is suggested further by the presence in their vascular wall of a muscularis, in distinct contrast to the absence of a muscularis in the smaller penetrating cerebral arteries and arterioles.The role of acidemia in the regulation of CBF in the perinatal animal requires further study. Whether produced by hypoxemia, lactate infusion, or respiratory means, acidemia caused a sharp increase in CBF in perinatal goats. However, effects were most impressive when acidemia was induced by elevation in PaCO 2 , a potent effector of CBF (as described earlier). Moreover, a subsequent study of the fetal lamb did not report an alteration in regional CBF over an arterial pH range from 6.9 to 7.5 produced by infusions of lactate or bicarbonate. Studies of newborn dogs and piglets showed inconsistent effects of lactate infusions or other changes in arterial pH on CBF.
A role for adenosine in regulation of CBF in the immature brain is suggested by observations, primarily in the neonatal piglet. Studies correlated CBF with parallel measurements of interstitial concentrations of adenosine and have used specific agonists and antagonists of the A 2 receptor (the adenosine receptor on vascular smooth muscle; the A 1 receptor, the adenosine receptor on neurons, is involved in decreasing glutamate release and Ca 2+ influx). The data suggest that adenosine has a vasodilatory effect and that it is involved in the cerebrovascular response to decreases in blood pressure and thereby cerebrovascular autoregulation. Recall that brain adenosine concentrations increase with hypoxia and seizures; both conditions require increases in substrate influx to brain. Finally, in addition to its vasodilatory effect, adenosine may influence CBF by inhibitions of platelet aggregation and activation of neutrophils (implicated in endothelial dysfunction), events shown to be important in the postischemic impairment of CBF in adult models.
Prostaglandins are important regulators of CBF in the perinatal period. a
a References .
Prostanoids may exhibit vasodilator or vasoconstrictor properties, depending on the specific prostanoid, and they appear to be important in the setting of both the upper and lower limits of autoregulation. However, in neonatal animals , prostanoids exert effects that are different from those observed in the adult. In general, these compounds function as cerebral vasodilators and are important in regulation of CBF with decreases in blood pressure within and below the autoregulatory range, with changes in blood PCO 2 and perivascular H + concentrations, and following ischemia, asphyxia, and seizures (conditions characterized by increases in cerebral prostaglandin biosynthesis). Prostaglandins also attenuate the vasoconstrictor responses of norepinephrine and are the apparent mediators of the vasodilatory responses of endogenous opiates. As a consequence of these important roles, indomethacin, through its inhibition of cyclooxygenase and thereby prostanoid biosynthesis, may have a variety of important cerebral hemodynamic effects that are vasoconstrictive. Such vasoconstrictor effects may be potentially beneficial (e.g., concerning prevention of intraventricular hemorrhage; see Chapter 24 ), or potentially deleterious (e.g., under conditions requiring maintenance or increase in CBF, as with hypotension, asphyxia, or seizures).Cerebral Blood Flow During and After Perinatal Asphyxia or Other Hypoxic-Ischemic Insults in Experimental Animal Models
Important cerebral circulatory effects of perinatal asphyxia and related hypoxic-ischemic insults have been defined by studies of a variety of experimental models, some based on techniques that result in impaired gas exchange between the mother and fetus or postnatally, and others based on controlled manipulation of only specific blood gases or of blood pressure. a
a References .
During asphyxia , three of these circulatory effects occur initially, and two occur with more prolonged episodes. The effects initially include (1) an alteration in the fetal circulation such that a larger proportion of the cardiac output is distributed to the brain, (2) an increase in total and regional CBF, and (3) a loss of vascular autoregulation, and, later, include (4) a diminution in cardiac output with the occurrence of systemic hypotension, and, largely as a consequence, (5) a decrease in CBF ( Table 13.4 ). After asphyxia , critical additional circulatory effects develop, and indeed from the clinical standpoint, these postinsult effects are as important, if not more so, than those occurring during asphyxia ( Table 13.5 ).↓ Glucose influx to brain |
↑ Glycogenolysis |
↑ Glycolysis |
↓ Brain glucose |
↑ Lactate production and tissue acidosis |
↓ Phosphocreatine |
↓ Adenosine triphosphate |
FETAL CONDITION | WHITE MATTER INJURY | BRAIN METABOLITE * | ||
---|---|---|---|---|
LACTATE | PHOSPHOCREATINE | ADENOSINE TRIPHOSPHATE | ||
Normoxic, normotensive | − | 3.2 | 0.7 | 0.7 |
Hypoxic, normotensive | − | 9.9 † | 0.5 | 0.9 |
Hypoxic, hypotensive | + | 19.5 † | 0.3 † | 0.1 † |
* Concentrations are mmol/kg; values are rounded off.
Redistribution of Fetal Circulation.
Promptly after the onset of asphyxia in the term fetal primate or lamb, cardiac output is redistributed such that a significantly larger proportion enters the brain, the coronary circulation, and the adrenals, at the expense of blood flow to other regions. a
a References .
Approximately twofold increases in the proportion of cardiac output to brain were noted in studies of term fetal primates. This redistribution of blood flow is reminiscent of the diving reflex observed in aquatic animals and appears designed to protect the most critical and vulnerable organs. The response requires an intact sympathoadrenal system. The important afferent components of the response include particularly the oxygen chemoreceptors. Moreover, to be effective, circulation must be maintained—hence the hypertension noted shortly after the onset of fetal asphyxia is particularly important.Increase in Cerebral Blood Flow.
The major purpose of the circulatory changes as outlined is to maintain CBF in the presence of impending tissue oxygen debt. In experiments with fetal and neonatal lambs, puppies, and primates, CBF in perinatal asphyxia increased generally by 50% to 500%. b
b References .
In severe and prolonged asphyxia, CBF eventually falls as a consequence of decreasing cardiac output (secondary to myocardial failure and hypoxic-induced bradycardia) and the loss of vascular autoregulation.The mechanisms underlying the initial increase in CBF relate in part to cerebral vasodilation, secondary to hypoxemia or hypercapnia, or both, presumably with increased perivascular H + concentration. Roles for elevated extracellular fluid concentrations of K + , adenosine, and prostaglandins, all of which increase markedly in brain with hypoxemia and ischemia, are likely. The particular importance of a rise or at least maintenance of blood pressure in the increase of CBF with asphyxia was indicated by several studies. In term fetal sheep subjected to asphyxia by cord compression, the initial increase in MABP persisted for 60 minutes before decreasing to normal values. Carefully controlled experiments with the same animal suggested that fetal blood pressure may be even more critical than local chemical factors, which lead to cerebral vasodilation, in the enhancement of CBF.
Although blood flow to various regions of the brain increases generally in concert with the increase in total CBF, distinct regional differences in this increase are apparent. In general, the increase in blood flow is most marked in brain stem structures and is least apparent in cerebral white matter. This general pattern was documented in the fetal lamb, neonatal lamb, and neonatal puppy. c
c References .
This effect has been interpreted as an attempt to maintain the integrity of vital brain stem centers. The mechanism for the heterogeneity in regulation of CBF is unknown; an endogenous opioid-mediated mechanism appears likely. Thus administration of naloxone results in an increase in telencephalic blood flow and oxygen metabolism and consequently a decrease in the fraction of CBF to the brain stem. This decrease in the fraction of flow to brain stem may impair the attempt to preserve vital brain stem centers. A likely conclusion from this work is that with hypoxia or asphyxia, the role of endogenous opiates is to suppress the cerebral rate of oxygen consumption, with the associated decrease in telencephalic blood flow serving to preserve the brain stem by an increase in the fraction of total brain blood flow to the brain stem. The burst in release of endogenous opiates with hypoxia and asphyxia and the well-known suppression of cerebral neural activity and oxygen consumption by endogenous opiates support this notion. In this context, administration of naloxone during asphyxia may be deleterious to the brain stem.Loss of Vascular Autoregulation.
A serious impairment of cerebral vascular autoregulation develops with perinatal asphyxia with the presence of a pressure-passive CBF. Using the radioactive microsphere technique and producing asphyxia (pH. 6.8 to 7.0) in term fetal sheep by partial occlusion of umbilical vessels, Lou et al. demonstrated a striking pressure-passive CBF. Marked hyperemia, with CBF values up to six times the normal value, occurred when MABP was raised to 60 to 70 mm Hg, whereas CBF declined to close to zero in large cortical areas when MABP was lowered to 30 mm Hg. Vascular autoregulation in these term fetal animals appeared to be very sensitive to asphyxia. The likely mechanism relates most probably to the hypoxemia and hypercapnia that are the hallmarks of perinatal asphyxia. The sensitivity of the autoregulatory system in the fetal and neonatal brain to these alterations in blood gas levels was described earlier (see the section on autoregulation). The implications of these data for ischemic injury to perinatal brain are obvious. In one study of near-term fetal sheep, autoregulation of CBF was lost within 4 minutes of cord occlusion, and overt cerebral injury occurred by 10 minutes secondary to decreased CBF in the presence of hypotension.
Hypotension and Diminished Cerebral Blood Flow.
Although the initial response to asphyxia is hypertension, this response is followed by hypotension. The rapidity and severity of this occurrence depend on the duration and severity of the asphyxial insult. In large part, this effect is related to a diminution in cardiac output, probably secondary to an effect on the myocardium. The consequence for the brain may be devastating, because the impairment of vascular autoregulation leaves CBF at the mercy of perfusion pressure. Deficits in CBF may be marked, with relatively modest changes in MABP. Impressive deficits in CBF (20% to 80%) have been demonstrated, particularly in the parasagittal regions of the cerebral hemispheres and especially posteriorly, in the term fetal monkey subjected to severe and prolonged asphyxia. A similar parasagittal distribution of cerebral cortical injury was demonstrated in near-term fetal sheep subjected to cerebral ischemia. The detailed regional study of newborn dogs demonstrated that cerebral white matter also is particularly likely to exhibit diminished blood flow with hypotension. In the preterm sheep (0.65 gestation), cerebral white matter was particularly affected with ischemia, with white matter injury then determined by the topographic distribution within the ischemic regions of vulnerable differentiating oligodendrocytes. These observations correlate well with the neuropathological and clinical observations made by asphyxiated human infants (see Chapters 16 and 20 ).
A summary of the major relationships between perinatal asphyxia and CBF during asphyxia is shown graphically in Fig. 13.12 . The initial effects leading to increased CBF are considered best as compensatory, adaptive responses (which could become maladaptive by leading to hemorrhage in vulnerable capillary beds). The later effects represent a decompensation of these responses and a cascade that leads to diminished CBF and brain injury.

Postasphyxial-Postischemic Effects.
The period after termination of the asphyxial-ischemic insult is critical because, during this interval, progression to brain injury occurs (see earlier discussion), and in the clinical setting, this time represents a window of opportunity for therapeutic intervention. The principal experimental models used have been near-term fetal sheep and neonatal piglets and rat pups, and the insults have primarily consisted of hypoxia-ischemia and, less commonly, asphyxia. a
a References .
The major circulatory effects identified are summarized in Table 13.5 . A consistent observation has been a marked increase in CBF on reperfusion, hyperemia that continues for up to several hours ( Fig. 13.13 ). This early increase in CBF is presumably related to the same mechanism operative for the initial increase in CBF during asphyxia noted earlier (i.e., the accumulation of such vasodilating factors as H + , K + , adenosine, and prostaglandins). Moreover, superoxide anion, a consequence of reperfusion after asphyxia (see earlier), may lead to a disturbance of cerebrovascular autoregulation through stimulation of vasodilation. This early increase in cerebral perfusion is followed by a decline toward the baseline. In some models, especially if associated with hypotension, CBF declines below normal with the threat of cerebral ischemia. This postasphyxial cerebral hypoperfusion has not been a consistent feature in all experimental models. It is likely that hypotension could lead to cerebral ischemia in this period, because autoregulation is not operative ( Fig. 13.14 ).

Importantly, a second (i.e., “delayed”) increase in CBF develops, with onset generally between 12 and 24 hours and with a duration of many hours or a day or more (see Fig. 13.13 ). This increase is associated with evidence of impaired mitochondrial oxygenation (as assessed by brain levels of oxidized cytochrome c), with the cellular energy failure, and with neuropathological evidence for neuronal and white matter injury. The delayed hyperemia, its association with energy failure, and its correlation with severity of brain injury have also been observed in asphyxiated human infants ( Chapter 18 , Chapter 19 , Chapter 20 ). The mechanisms underlying the vasodilation and hyperemia are not established but may relate in part to NO. Because NO synthesis in endothelial cells (eNOS) is activated after hypoxic-ischemic insults, an attempt to reduce the delayed cerebral hyperemia with an NOS inhibitor was attempted in the fetal sheep model of ischemia. The inhibitor did attenuate the delayed hyperemia, but surprisingly, inhibition of NO synthesis increased histological injury. This combination of findings suggests that NO synthesis has a protective effect either because of the vasodilatory effect or because of a biochemical effect.
Cerebral Blood Flow in the Human Newborn
Methodology
In approximately the past 25 to 30 years, considerable insight into CBF in the human newborn has been provided by application of one or more of several techniques ( Table 13.6 ). The largest amount of information has been provided by the xenon-133 clearance technique . The technique uses administration of xenon-133, either by intraarterial or intravenous injection or by inhalation (preferably intravenous injection), and detection of the brain clearance of xenon-133, specifically the gamma radiation thereof, by external detectors. The particular advantage of the xenon-133 clearance technique is the ability to provide quantitative data with relatively low radiation exposure and portable equipment. Xenon computed tomography has the advantage of providing regional data, but the technique requires transport to a specialized suite.
EXPERIMENTAL CONDITION | BRAIN CONCENTRATION (µmmol/g) | |
---|---|---|
ADENOSINE TRIPHOSPHATE | LACTATE | |
Control | 2.2 | 3.0 |
Circulatory arrest | 0.2 | 13.0 |
Circulatory arrest and glucose pretreatment | 0.3 | 33.0 |
Positron emission tomography (PET) has been valuable in demonstration in the premature and term newborn of normal values of regional CBF, coupling with oxygen consumption, increases of flow with seizure, and characteristic changes in premature infants with periventricular hemorrhagic infarction and intraventricular hemorrhage, as well as in term asphyxiated infants with parasagittal cerebral injury. The particular advantage of PET is the ability to provide not only quantitative data but also regional information. Single photon emission tomography also provides regional data but is nonquantitative. Near-infrared spectroscopy , a noninvasive optical technique, has the capability to provide serial quantitative measurements of CBF and is discussed in detail in Chapter 10 . With venous occlusion plethysmography , changes in intracranial volume after brief occlusion of the jugular veins are determined by a strain-gauge instrument placed around the compliant infant skull. This technique cannot provide quantitative information and has the disadvantage of causing a transient rise in intracranial pressure. Application of this method is discussed briefly later. The Doppler ultrasonic technique for measurement of CBF velocity, a noninvasive method, can provide serial information about cerebrovascular resistance and flow velocity in the insonated cerebral vessels. Determination of changes in volumic flow from the velocity data is complicated by the inability to determine the cross-sectional diameter of the insonated vessel. Electrical impedance techniques are noninvasive but have not proven sufficiently sensitive to be consistently useful. MR techniques for determination of CBF are under intensive study in the human newborn infant, either after administration of a paramagnetic contrast agent (e.g., gadolinium) or by arterial spin labeling (ASL) perfusion-weighted MRI ( Fig. 13.15 ). ASL perfusion-weighted MRI is the only approach that enables direct and noninvasive measurements of CBF in different brain regions, without the need to inject contrast material or expose the newborn to ionizing radiation. To date, a handful of studies have demonstrated the feasibility of using ASL in newborns. In addition, a few recent studies have demonstrated the benefit of combining timely measurements of CBF by ASL-MRI with other modalities, such as near-infrared spectroscopy or MR spectroscopy, to better assess changes in cerebral perfusion, metabolism, and oxygenation in sick newborns. The method has been used effectively in studies of CBF with human brain maturation and in several neonatal pathological states (i.e., hypoxic-ischemic encephalopathy, arterial stroke, and congenital heart disease; see Chapters 20 and 21 ).

Development and Normal Values of Cerebral Blood Flow in the Human Newborn
Changes Immediately After Delivery.
A sharp decrease in “apparent” CBF (“apparent” because the plethysmographic method is only semiquantitative) occurs in the term infant in the first hours after delivery ( Fig. 13.16 ). The decrease in the first 3 hours is nearly twofold, and over the ensuing hours, CBF is relatively stable. The reason for the relatively higher value shortly after delivery is not known, although a relationship with higher PaCO 2 levels immediately after birth has been suggested. Alternatively, it is possible that a reflex activity, mediated by vagal afferents, is operative because the relatively higher CBF near the time of birth requires intact vagus nerves in the sheep. Both factors may be relevant in the human newborn, when PaCO 2 levels may be elevated and vagal activity from lung expansion may be considerable. A third factor may involve arterial oxygen content, because, as noted earlier, a similar sharp decline in CBF after birth has been observed in the lamb and correlates well with the increase in arterial oxygen content in the newborn versus fetal state. The relatively enhanced CBF in the first minutes to hours after delivery may provide a margin of safety for cerebral metabolic needs in the period of adaptation to birth.

Changes Beyond the Immediate Postpartum Period.
Data obtained by PET suggest that CBF is approximately 20% of the adult value in the premature infant of approximately 28 weeks of gestation and approximately 40% of the adult value in the term newborn. Serial studies of CBF in normal preterm infants show an approximately twofold increase in flow over the first 3 days of life, perhaps related to an increase in cardiac output. One study of preterm infants by spatially resolved near-infrared spectroscopy showed a sharp increase in cerebral oxygenation during this period, most consistent with an increase in CBF. Doppler studies of CBF velocity also are consistent with this postnatal increase in CBF (see Chapter 10 ).
Normal Values.
Values for CBF reported in the human premature newborn, studied by xenon clearance and shown in Table 13.7 , are generally between 10 and 20 mL/100 g per minute. A similar range is apparent in studies by PET and near-infrared spectroscopy. The correlations with the findings in developing animals described earlier are obvious. Regional values for CBF are notable for higher flows in cerebral gray matter structures than in cerebral white matter.
EXPERIMENTAL CONDITION | PHOSPHOCREATINE * | ATP * | LACTATE * |
---|---|---|---|
Control | 3.00 | 2.41 | 1.6 |
Ligation-hypoxemia † | |||
Saline (60 min) | 1.00 | 1.25 | 11.1 |
Glucose (60 min) | 1.80 | 2.31 | 15.2 |
Saline (120 min) | 0.35 | 0.43 | 9.4 |
Glucose (120 min) | 1.00 | 1.80 | 25.5 |
* Values are mean concentrations (mmol/kg) in hemisphere ipsilateral to carotid ligation.
† All values for ligated-hypoxemic animals different from controls ( P < .05), and all values for glucose-treated animals different from saline-treated animals ( P < .05).
Regulation in the Human Newborn.
The major established regulatory mechanisms for CBF in the human newborn include autoregulation, PaCO 2 , oxygen delivery, blood glucose, and neuronal activity (e.g., seizure). Certain pharmacological agents also have been shown to exert regulatory effects. These various regulatory factors and their effects on CBF are summarized in Table 13.8 and are reviewed briefly next.
Glucose transport into the brain and glucose concentration in the brain are increased. Lactate levels are increased, and intracellular pH values are decreased but recover promptly. |
Decrease in the cerebral metabolic rate of oxygen is prevented, perhaps reflecting improved mitochondrial function. |
Improvement in high-energy phosphate levels is usual but has not reached statistical significance in all studies. |
Neuropathological injury may be prevented, ameliorated, or accentuated, according to the model of hypoxia-ischemia and the species and state of maturation of the animal. |
Improved survival occurs and may relate at least partially to improvement in cardiorespiratory function. |
Determinations of cerebral lactate and high-energy phosphates, as a function of blood glucose, are needed in asphyxiated human newborns for definitive recommendations concerning glucose supplementation. |
Autoregulation.
Autoregulation appears to be operative in both the normal human preterm and full-term infants. a
a References .
Although the actual limits of the autoregulatory plateau cannot be established with certainty , the approximate autoregulatory range appears to be from 25 to 50 mm Hg MABP. Both the size of this range and its actual upper and lower limits vary according to gestational age ( Fig. 13.17 ) and, likely, postnatal age and multiple other factors. Autoregulation in mature animals and adult humans is rendered inoperative by factors that lead to pronounced vasodilation (e.g., hypercarbia, hypoxia, hypoglycemia, seizure, postasphyxial state, and selected cytokines), and available data suggest that these factors also impair autoregulation in the human infant, particularly the seriously asphyxiated full-term infant and in the sick, mechanically ventilated preterm infant (see Table 13.8 ). bb References .

The problem of a persistent pressure-passive cerebral circulation is particularly important in sick premature infants. Thus initial studies using the invasive technique of radioactive xenon clearance initially showed that certain premature infants, mechanically ventilated and usually clinically unstable, appeared to exhibit pressure-passive cerebral circulation. This fundamental initial observation has been confirmed by less invasive methods multiple times. Thus, in such sick premature infants with pressure-passive cerebral circulation, it would be expected that when blood pressure falls, as occurs commonly in such infants, so would CBF, with the consequence of cerebral ischemia. The presence of arterial end zones and border zones and vulnerable early differentiating oligodendroglia in developing cerebral white matter would render this region especially vulnerable (see Chapter 15 ). Moreover, the particular danger is compounded by the very low normal blood flow to cerebral white matter in the premature infant, a feature suggesting that a minimal margin of safety may exist. In one serial study of 32 mechanically ventilated premature infants from the first hours, near-infrared spectroscopy was used to demonstrate a pressure-passive cerebral circulation in 53%. The example shown in Fig. 13.18 is typical (i.e., an infant with MABPs that fluctuate gradually over minutes and are accompanied by parallel changes in the cerebral circulation). The nadirs of blood pressure often are not markedly low and thus may be readily overlooked . However, the cumulative effect of many repeated modest declines in CBF is likely to be considerable (see Chapter 15 ). Importantly, nearly all the cases of PVL (and severe germinal matrix hemorrhage/intraventricular hemorrhage) later identified in the series of 32 infants were in the pressure-passive group. These findings were critical because they suggested that (1) infants with a pressure-passive cerebral circulation could be identified by near-infrared spectroscopy before the occurrence of white matter injury, (2) that the circulatory abnormality is related strongly to the occurrence of white matter injury, and (3) if the pressure-passive state could be corrected, perhaps the white matter injury could be prevented. However, this study involved a relatively small number of infants.

A subsequent study of 90 premature infants used a frequency-based assessment of autoregulation and quantitated the degree and duration of altered autoregulation over the first 5 days of life. Pressure-passive epochs were documented in 95% of the infants . The overall mean proportion of the pressure-passive time was 20%, although some infants had a pressure-passive state more than 50% of the time. The likelihood of a pressure-passive state increased with decreasing gestational age and periods of hypotension.
More recently, using near infrared spectroscopy (NIRS) methodology, high coherence between tissue oxygenation index (TOI) and MABP was also observed with low blood pressure in the sickest infants, suggestive of a narrow window for autoregulation in such sick immature infants. Additional technical analysis with time domain analysis described the cerebral oximetry index (COx) as the moving and linear correlation coefficient between slow waves of MABP and regional cerebral oxygen saturation (rSO 2 ). Researchers observed that lower MABP during the first 3 days of life was associated with impaired autoregulation (COx >0.5) in preterm infants at ≤30 weeks of gestational age. There has been debate regarding whether the two methods are equivalent or one is superior to the other; however, in a series of 60 preterm infants, time-domain analysis appeared more robust compared with coherence function analysis.
A further index of cerebral vascular reactivity is a correlation coefficient of TOI and MABP (TOx) with HR (tissue oxygenation heart rate, TOHRx), believed to improve the reflection of perfusion with both blood pressure and heart rate. Using this measure in 31 preterm infants at median gestational age of 26 weeks, TOHRx was significantly correlated with gestational age ( R = −0.57, P = .007), birth weight ( R = −0.58, P = .006), and the Clinical Risk Index for Babies II ( R = 0.55, P = .0014)—a prediction score of mortality and morbidity in the preterm infant. Defining “optimal” MABP by the strength of cerebral autoregulation has been studied in adults with traumatic brain injury. By measuring spontaneous fluctuations in cerebral perfusion pressure, it is possible to calculate the range of perfusion pressure where autoregulation is strongest. In one study, patients were more likely to have a favorable outcome when cerebral perfusion pressure was close to “optimal.” Recently, continuous monitoring of cerebral vascular reactivity in preterm infants, with TOHRx, has been used to define values of MABP OPT where cerebral vascular reactivity is strongest. Preterm infants who died had a higher mean absolute deviation from MABP OPT than patients who survived. Furthermore, deviation of MABP above the optimal values was observed in infants who had worse germinal matrix-intraventricular hemorrhage grades. (There remain significant technical challenges in obtaining reliable measurements of MABP OPT at the cot side. For example, a minimum of 2 to 4 hours of continuous artifact-free data is necessary to create a reliable U -shaped autoregulatory curve with spontaneous fluctuations in heart rate and TOI. Currently all descriptions of MABP OPT have been undertaken on retrospective analysis of data.)
The lower limit of the autoregulatory curve in newborns obviously is of great importance, particularly for management purposes and especially in sick premature infants. The precise lower limit remains unknown, and it is likely that this value varies not only with gestational age but also with postnatal age and with factors that alter the concentration of vasoactive molecules in brain (e.g., blood gases, cytokines, seizures, hypoxia-ischemia). In one report, blood pressure values in the first day of life in infants 24 to 30 weeks of gestation of less than 31 mm Hg were associated with impaired electroencephalogram (EEG) continuity on the EEG, a finding raising the possibility of decreased CBF at such values. Similarly, in a study of extremely low-birth-weight infants (mean birth weight 772 g, mean gestational age 26 weeks) by near-infrared spectroscopy, a pressure-passive cerebral circulation resulted when MABPs were lower than 30 mm Hg. However, to what extent, if any, arterial blood pressures lower than 30 mm Hg are clearly dangerous is unclear. Thus a careful study of cerebral electrical activity and cerebral fractional oxygen extraction, presumably a reflection of CBF, in 35 23- to 30-week premature infants showed no electrical abnormality at mean blood pressure levels higher than 23 mm Hg.
Carbon Dioxide.
PaCO 2 is a potent regulator of CBF in the human newborn. a
a References .
The marked reactivity of CBF to PaCO 2 is present in the first hours of life in spontaneously breathing preterm infants but does not appear until the second day in mechanically ventilated preterm infants ( Table 13.9 ). The reason for the attenuated reactivity of CBF to PaCO 2 in the first day of life in mechanically ventilated preterm infants is unclear, although the same phenomenon has been observed in the newborn monkey, rat, dog, and lamb. A state of attenuated or absent reactivity to PaCO 2 , as with blood pressure, has been observed both in seriously asphyxiated full-term infants and in mechanically ventilated preterm infants before severe intracranial hemorrhage ( Table 13.10 ; see Chapter 14 , Chapter 15 , Chapter 16 , Chapter 18 , Chapter 19 , Chapter 20 ). In general, the loss of reactivity to PaCO 2 follows the loss of autoregulation (but precedes loss of reactivity to hypoxemia). In one report, a progressive loss of cerebrovascular autoregulation was noted with increasing PaCO 2 ≥45 mm Hg.Lower rate of energy utilization |
Lower rate of accumulation of toxic products (i.e., lactate) |
Utilization of lactate and ketone bodies for energy |
METABOLIC COMPOUND | TIME AFTER DELIVERY (MIN) | ||
---|---|---|---|
1 | 10 | 60 | |
PERCENTAGE OF TERM FETAL VALUES | |||
Glycogen | 88 | 74 | 90 |
Lactate | 367 | 408 | 230 |
Lactate-pyruvate | 425 | 181 | 157 |
Phosphocreatine | 38 | 105 | 170 |
Adenosine triphosphate | 67 | 92 | 96 |
The mechanism for the vasodilating effect of carbon dioxide relates to the increase in perivascular H + concentration , as observed in experimental models. The observation of a 50% decrease in CBF after sodium bicarbonate administration to term and premature infants with acidosis also supports the important role of perivascular H + concentration. The mechanism proposed for a decrease in the latter situation was enhanced movement of bicarbonate across the blood-brain barrier “because of vasodilation caused by the asphyxia” in these infants. The demonstration of a decrease in CBF after sodium bicarbonate administration in acidotic postasphyxial infants may have important implications for management. Sodium bicarbonate is no longer recommended for use in the resuscitation of the term or preterm newborn infant due to its association with an increased mortality and morbidity.
Oxygen.
Arterial oxygen concentration is an important effector of CBF in the human infant, as it is in the perinatal animal. a
a References .
A vivid demonstration of the vasoconstrictive effect of oxygen is the observation that preterm infants administered 80% oxygen during stabilization at birth had a 23% lower value for CBF than infants administered room air during stabilization, when measured by xenon-133 clearance at 2 hours of life. This finding may have implications concerning the use of high concentrations of inspired oxygen at the time of birth. The administration of 100% oxygen for resuscitation of the term newborn infant has been associated with a markedly increased risk for death and has led to the clear recommendation that all term-born infants be resuscitated in room air. Arterial oxygen concentration is related not only to PaO 2 but also to hemoglobin concentration and the oxygen affinity of hemoglobin. In preterm infants studied in the first day of life, Pryds and Greisen observed a mean increase in CBF of 11.9% per 1 mmol/kg decrease in hemoglobin concentration. In a separate series of preterm infants studied at a mean postnatal age of 3.7 weeks, a mean increase in CBF of 5% per percentage point of decrease in hematocrit was documented. Oxygen delivery to the brain may be affected not only by hemoglobin concentration but also by the viscosity of blood, at which point the inverse relationship of CBF with hemoglobin concentration becomes more pronounced. However, in general, hematocrit does not alter blood viscosity in the newborn at levels lower than approximately 60% (and perhaps somewhat higher). Finally, a direct relationship of CBF (determined by xenon-133 clearance) with the relative proportion of fetal hemoglobin has been shown, presumably reflecting the stronger affinity of fetal hemoglobin for oxygen. This conclusion had been suggested by a prior study of CBF velocity.Glucose.
A striking observation by Pryds et al. established an important role for glucose in regulation of CBF in the human newborn (see Table 13.8 ). As discussed in more detail in Chapter 25 , an increase in CBF became apparent as blood glucose concentration decreased to less than approximately 30 mg/dL (1.7 mmol/L). Increases in CBF of twofold to threefold then occurred in proportion to the decline in blood glucose. The mechanism for this vasodilatory effect of glucose is not clear, but stimulation of beta-receptors by the increased compensatory secretion of epinephrine is suggested by data in human adults. The clinical significance of this effect of glucose could be appreciable (see Chapter 25 ).
Neuronal Activity (Seizure).
The coupling of neuronal activity to CBF is apparent in at least two situations: sleep states and seizure. A decrease in CBF during sleep has been shown by xenon-133 clearance. The effect is not striking. A striking increase (≈50%) in CBF with the excessive neuronal activity of seizure has been documented in the human newborn by PET (see Chapter 12 ). This effect had been suggested by earlier studies of CBF velocity by Doppler.
Pharmacological Agents.
Indomethacin and aminophylline are the two pharmacological agents shown to have a clear effect on CBF in the human newborn. Indomethacin administration in doses used for closure of the ductus arteriosus led to a 20% to 40% decrease in CBF in the premature infant, as studied by xenon-133 clearance and near-infrared spectroscopy. Notably, however, no change in CBF, assessed by Doppler, was observed after continuous versus bolus infusion of indomethacin. The vasoconstrictive effect is mediated by the inhibition of synthesis of vasodilatory prostaglandins at the cyclooxygenase step, as shown in experimental models and studies of isolated neonatal human cerebral artery. An increase in cerebrovascular resistance was shown by Doppler studies of very low-birth-weight infants after indomethacin administration. Interestingly, by contrast with indomethacin, ibuprofen does not lead to a decline in CBF.
Administration of aminophylline , an antagonist of adenosine, led to only a small decrease (10% to 15%) in CBF in the human premature infant within 1 hour of intravenous administration. No alteration of visual evoked potentials accompanied this modest decrease in blood flow. The use of caffeine in the preterm infant has resulted in similar observations of a reduction in CBF. In a study of 40 preterm infants after a loading dose of caffeine using Doppler cerebral sonography, cardiac echocardiography, and cerebral spatially resolved near-infrared spectroscopy, mean anterior cerebral artery peak and time average mean blood flow velocity fell significantly (by 14% and 17.7%, respectively) at 1 hour post-caffeine loading dose. Cerebral TOI fell from predose levels by 9.5% at 1 hour, with partial recovery to 4.9% reduced at 4 hours post dose. There were no significant changes in left or right ventricular output, transcutaneous oxygen saturation, transcutaneous PCO 2 , or total vascular resistance. Dopamine administered to treat hypotension was reported in two studies to increase CBF as well as arterial blood pressure. However, a more recent study of preterm and term piglets showed no increase of CBF with dopamine or dobutamine.
Perinatal Asphyxia, Autoregulation of Cerebral Blood Flow, and Cerebral Hyperemia in the Human Newborn Infant
Impaired Autoregulation.
A xenon-133 study of CBF in a group of 19 term and preterm infants first suggested that autoregulation in the human newborn is very sensitive to perinatal asphyxia. Thus 19 infants were examined with the xenon clearance technique “a few hours after birth.” Eleven of these infants weighed less than 2000 g. Although most of the infants were considered “distressed,” Apgar scores at 5 minutes were less than 7 in only 4 of the 19 infants. At the time of study, pH was less than 7.20 in only 4 infants. For the total group of 19 infants, a linear relationship existed between CBF and systolic blood pressure ( Fig. 13.19 ). This pressure-passive relationship suggests inoperative vascular autoregulation and was seen to a similar degree in the infants less than or more than 2000 g body weight. This apparent impairment of vascular autoregulation is directly reminiscent of the data obtained with fetal and neonatal animals after asphyxia (see the earlier section).

Impaired Vascular Reactivity and Cerebral Hyperemia.
Subsequent work clarified the relationship between perinatal asphyxia and the impairment of vascular reactivity—particularly autoregulation. In a systematic study of 19 term infants (mean birth weight, 3200 g) with perinatal asphyxia defined by a 5-minute Apgar score of 5 or lower and umbilical cord pH lower than 7.0, or both, a striking relationship among the severity of brain injury, the absolute value of CBF, and the reactivity to changes in blood pressure and PaCO 2 was defined (see Table 13.10 ). Thus infants with the poorest neurological outcome (isoelectric amplitude-integrated EEG, death) had the highest values for CBF and no autoregulation or carbon dioxide reactivity (see Table 13.9 ). Infants with a burst-suppression EEG and moderate to severe brain injury had slightly elevated values for CBF and impaired autoregulation but retained reactivity to PaCO 2 (see Table 13.10 ). Infants without evidence of brain injury had normal values for CBF, intact autoregulation, and reactivity to PaCO 2 . A later study of 16 term infants with hypoxic-ischemic encephalopathy used PET to determine CBF primarily at 1 to 4 days of life and found higher flows in those infants with abnormal neurological outcome (35.6 mL/100 g per minute) than in those with normal neurological outcome (18.3 mL/100 g per minute).
The pronounced, sustained cerebral hyperemia observed in the human infant has been shown by less invasive techniques, such as Doppler ultrasound and near-infrared spectroscopy. Thus determinations of CBF velocity in term infants with hypoxic-ischemic encephalopathy from approximately 6 to 130 hours after the insult showed an increase in mean flow velocity with decreased resistance indices (i.e., vasodilation). Similarly, studies of asphyxiated human infants on the first day of life by near-infrared spectroscopy are consistent with a loss of vascular reactivity and an increase in cerebral blood volume and CBF, with temporal characteristics similar to those observed in fetal sheep, described earlier. The mechanism for this hyperemia is unclear. An increase in neuronal excitability, although documented after hypoxic-ischemic insults, seems unlikely in view of the relation of highest flows to isoelectric EEG. It appears more likely that an accumulation of vasodilatory compounds or vascular injury, or both, occurs and that this accumulation or injury in many ways may be similar in the human newborn and the perinatal animal. Delineation of the mechanisms underlying this vasoparalytic state and cerebral hyperemia after perinatal asphyxia in the human infant will be of major importance and may be able to be further explored with magnetic resonance techniques such as ASL.
The aforementioned data thus define in the postasphyxial human newborn a state of vasoparalysis and cerebral hyperemia that is correlated with the degree of brain injury and presumably the severity of the asphyxial insult. The altered vascular reactivity, with autoregulation impaired more readily than carbon dioxide reactivity, is similar to observations made in the postasphyxial state in perinatal animal models (see earlier discussion). Presumably, a state of maximal vasodilation exists, related perhaps to the effects of elevated perivascular H + concentration, prostaglandins, adenosine, free radicals, or NO, or all these factors. Whether this hyperemic state is caused by the same factors that lead to the brain injury, is an adaptive mechanism to preserve brain tissue, or in some way causes additional brain injury remains to be clarified. It does appear likely that the loss of vascular reactivity renders the infant vulnerable to systemic hypotension and resulting cerebral ischemia.
Biochemical Mediators of Hypoxic-Ischemic Cerebral Injury—Human Newborn and Relevant Experimental Models
Normal Carbohydrate and Energy Metabolism
Because glucose and oxygen are the principal driving forces for energy production in brain, the major initial biochemical effects of well-established oxygen deprivation are exerted at the levels of carbohydrate and energy metabolism. A brief review of these areas of metabolism is appropriate here (see also Chapter 25 ). Detailed discussions are available from other, more specialized sources.
Glucose Uptake
Glucose from blood is taken up by the brain through a process of carrier-mediated, facilitated diffusion that allows for the transport of glucose faster than would be expected by simple diffusion. Specific glucose transporter proteins are involved (see Chapter 25 ). The transport process, however, is not energy-dependent and thus differs in that critical fashion from active transport. Glucose transport across the blood-brain barrier uses the heavily glycosylated form of the facilitative glucose transporter protein, GLUT1 (55 kDa). Transport across glial membranes is facilitated by the lower molecular form of GLUT1 (45 kDa), and transport across the neuronal membrane is facilitated by GLUT3. The levels of these proteins are relatively low in the immature brain and are limiting to glucose transport and utilization. Consistent with the experimental findings, elegant studies of human infants by PET show that the cerebral metabolic rate for glucose in the brain of preterm newborn infants is approximately one-third of that in the brain of adults and that this difference relates to a diminished transport capacity rather than a diminished affinity of the transporters for glucose.
Formation of Glucose-6-Phosphate
Glucose in the brain is phosphorylated to glucose-6-phosphate; the enzyme involved is hexokinase ( Fig. 13.20 ). The activity of hexokinase is linked to glucose uptake by the cell and is inhibited by the product of the reaction, glucose-6-phosphate. The activity of this enzyme is also lower in the neonatal versus adult rat brain. Glucose-6-phosphate is a pivotal metabolite in glucose metabolism, with three major fates: (1) glycolysis and, ultimately, energy production; (2) glycogen synthesis; and (3) the pentose monophosphate shunt for synthesis of lipids (by formation of reduced nicotinamide adenine dinucleotide phosphate [NADPH]) and nucleic acids.

Glycogen Metabolism
Glycogen is found in relatively small concentrations in the brain but represents an important storage form of carbohydrate. Glycogen synthesis and degradation occur primarily in astrocytes . Glycogen synthesis proceeds through glucose-1-phosphate and then to glycogen through glycogen synthetase. Glycogen breakdown to glucose-1-phosphate through phosphorylase, and then to glucose-6-phosphate through phosphoglucomutase, is an important mechanism for generating oxidizable substrate by the glycolytic pathway (see Fig. 13.20 ). Glycogen in astrocytes provides fuel to neurons first by conversion to lactate and then transport of lactate by specific monocarboxylate transporters to neurons. By a similar mechanism, astrocytes degrade glycogen to lactate that is provided to developing oligodendrocytes , primarily for lipid biosynthesis. Brain phosphorylase is activated by cyclic adenosine monophosphate (AMP), and levelsof cyclic AMP are elevated by certain hormones, such as epinephrine. Epinephrine release is accentuated sharply with hypoxic, ischemic, and asphyxial insults. Although glycogen is broken down in the perinatal brain under certain circumstances, the capacity of the perinatal degradative system, at least in the rodent brain, is considerably less than that in the adult.
Glycolysis
The major portion of glucose-6-phosphate enters the glycolytic pathway to result ultimately in the formation of pyruvate. The major control step involves the conversion of fructose-6-phosphate to fructose-1,6-diphosphate; the rate-limiting enzyme involved is phosphofructokinase (see Fig. 13.20 ). The major mechanism of control of this enzyme is through allosteric effects—involving conformational changes of component peptides—and thus it is very rapid in onset. The activity of phosphofructokinase is inhibited by ATP, phosphocreatine (PCr), and low pH and activated by adenosine diphosphate (ADP), inorganic phosphorus (Pi), cyclic AMP, and ammonium ion.
Under aerobic conditions, the major product of glycolysis is pyruvate, which enters the mitochondrion and is converted through the pyruvate dehydrogenase complex to acetyl coenzyme A (acetyl-CoA; see Fig. 13.20 ). This mitochondrial enzyme is inhibited by an increase in the ATP/ADP ratio and is activated by a decrease in this ratio. Acetyl-CoA is used for fatty acid and cholesterol biosynthesis and for acetylcholine synthesis, but particularly for entry into the citric acid cycle for energy production.
Citric Acid Cycle and Electron Transport Chain
Mitochondrial acetyl-CoA enters the citric acid cycle and undergoes oxidation to carbon dioxide (see Fig. 13.20 ). The rate-limiting step is the conversion of isocitrate to alpha-ketoglutarate, catalyzed by the enzyme isocitrate dehydrogenase. A critical allosteric regulator of this enzyme is the ratio of ATP to ADP; an increase in the ratio causes a decrease in activity of the cycle, and a decrease in the ratio causes an increase in activity of the cycle. The electrons or reducing equivalents (reduced nicotinamide adenine dinucleotide [NADH], flavin adenine dinucleotide [FADH]) generated by the citric acid cycle next enter the electron transport system.
The transport of electrons takes place through a multimember chain of electron carrier proteins and is associated with release of free energy, which is used to generate ATP from ADP and Pi. The free energy, in essence, is “captured” in this high-energy phosphate bond. ATP is generated at three steps in the scheme, and because the final electron acceptor is oxygen, the process is called oxidative phosphorylation . Molecular oxygen is reduced, and water is the final product formed. The ATP generated by the citric acid cycle and the electron transport system is transported from the mitochondrion by a specific carrier and ultimately is used in the brain primarily for transport processes (especially of ions and neurotransmitters for impulse transmission and for the prevention of dangerous increases thereof; e.g., extracellular glutamate, cytosolic Ca 2+ ) and for synthetic processes (especially of neurotransmitters, but also lipids and proteins, particularly in the developing brain). The principal ions involved in ATP consumption are sodium (Na + ), potassium (K + ), and Ca 2+ ; in the adult brain (under normal conditions), approximately 60% to 75% of ATP is used for maintenance of membrane gradients of these three ions, especially Na + and K + .
Summary
The concerted action of glycolysis, the citric acid cycle, and the electron transport system, operative under aerobic conditions, results in the formation of 38 molecules of ATP for each molecule of glucose oxidized ( Fig. 13.21 ). The glycolytic portion of the pathway occurs in the cytosol and generates only 2 of the 38 molecules of ATP; the bulk of the ATP is generated in the mitochondrial portion of the pathway, which begins with pyruvate. The ATP generated is transported from the mitochondrion by a specific carrier and is used in the brain for two major purposes: transport and synthetic processes . Quantitatively, the most important transport processes involve ions in neurons for impulse transmission and maintenance of Ca 2+ homeostasis. Synthetic processes are important in the developing brain and involve neurotransmitters, structural and functional proteins, and membrane lipids.

Effects of Hypoxemia on Carbohydrate and Energy Metabolism
Major Changes
Hypoxemia is accompanied by numerous effects on carbohydrate and energy metabolism in the brain ( Table 13.11 ), effects that are understandable when viewed in the context of the normal metabolism just reviewed. Although it is likely that lack of oxygen is the major pathogenetic factor in these changes, it is difficult to produce hypoxemia experimentally without also causing other major metabolic changes that either accompany the hypoxemic insult or occur as a consequence of the insult (e.g., hypercapnia, acidosis, and hypotension). In most studies, however, these other changes either are prevented or are documented.
↑ Glucose influx to brain |
↑ Glycogenolysis |
↑ Glycolysis |
↓ Brain glucose |
↑ Lactate production and tissue acidosis |
↓ Phosphocreatine |
↓ Adenosine triphosphate |
The quantitative and temporal aspects of the biochemical changes associated with a severe hypoxemic or anoxic insult (i.e., nitrogen breathing) in the newborn mouse are depicted in Figs. 13.3 to 13.5 . The earliest significant changes are a decrease in brain glycogen, an elevation in lactate, and a decrease in PCr. These are followed by a decrease in brain glucose and, finally, ATP. The changes appear to reflect principally the impaired production of high-energy phosphate, secondary to failure of the coupled mitochondrial system of the citric acid cycle and electron transport chain—in turn, a consequence of the lack of the ultimate electron acceptor, oxygen. In response to the anaerobic state, glycolysis becomes the sole source of ATP production, and because lactate is the principal product of anaerobic glycolysis, only two molecules of ADP are generated for each molecule of glucose metabolized ( Fig. 13.22 ). This number is clearly a serious difference from the 38 molecules generated under aerobic conditions (see Fig. 13.21 ). Glycolysis is accelerated 5- to 10-fold, and an attempt to meet this enhanced need for glucose is made by a combination of glycogenolysis and increased net uptake of glucose from blood. (Glycogen contributes approximately one third of the cerebral energy supply under these conditions. ) Despite this acceleration, brain energy demands cannot be met, and ATP levels begin to fall after 2 minutes and decrease by 30% after6 minutes.

The relationship between arterial oxygen delivery and brain PCr levels (expressed as the ratio of PCr to Pi determined by MR spectroscopy) has been clarified in studies of the neonatal dog. Thus a crucial threshold decrease in the PCr/Pi ratio of 50% occurred when arterial oxygen pressure decreased to 12 mm Hg (approximate arterial oxygen saturation, 20%). The importance of the 50% decrease in PCr/Pi ratio relates to the finding in the neonatal piglet that at this level, brain lipid peroxidation and impaired Na + /K + -ATPase activity occur. The critical value of arterial partial pressure of oxygen (PaO 2 ) required to lead to the 50% decline in the PCr/Pi ratio in the neonatal dog (12 mm Hg) was higher at 7 to 21 days (17 mm Hg) and higher in the adult (23 mm Hg). This lower threshold value of PaO 2 in the neonatal animal correlated with in vitro data showing more efficient oxygen extraction in the neonatal animals (see later discussion). At any rate, it is clear that marked hypoxemia is required to produce serious changes in the brain energy state in the neonatal animal.
Maturational Vulnerability.
Studies of the effect of hypoxemia on brain energy metabolism in the immature rat brain have delineated a particular window of vulnerability. Thus the most marked declines in PCr and nucleoside triphosphates, defined by MR spectroscopy, occurred in the second postnatal week. This period of heightened vulnerability corresponds with the period of maximal susceptibility to excitotoxic neuronal injury and to epileptogenic effects of hypoxemia, as well as with the period of maximal expression of specific excitatory amino acid receptors, incomplete maturation of inhibitory transmission, relatively low levels of Ca 2+ binding proteins, and incomplete maturation of Na + /K + -ATPase levels (see later discussion). Taken together, these data suggest that the vulnerability of the immature rat in the second versus the first week of life relates to the increased propensity to develop with hypoxia, a hyperexcitable, hypermetabolic state in neurons, which leads to more marked declines in high-energy phosphates because of increased utilization. These considerations could help explain the greater likelihood of neuronal injury with hypoxia in the term brain than in the premature brain of the human.
Regional Vulnerability.
Studies in the newborn dog defined the regional changes in glucose and high-energy metabolism. Thus animals subjected to acute hypoxemia (oxygen pressure [PO 2 ] ≈ 12 mm Hg) and studied by the autoradiographic 2-[ 14 C]deoxyglucose technique exhibited increased glucose utilization in most gray matter structures and every white matter structure. Moreover, the degree of hypoxemia was sufficient to cause the accumulation of lactate in the brain in both gray and white matter, but only in white matter did a decline in energy state occur ( Table 13.12 ). Thus it appears that anaerobic glycolysis with its accelerated glucose utilization was capable of preserving the energy state in gray matter but not in white matter . Moreover, the finding that glucose levels declined more drastically in white matter than in gray matter (see Table 13.12 ) suggests that glucose influx could not meet the increased demands for glucose in white matter. That the rate of glucose metabolism, in fact, was limited by glucose influx from blood is supported by the demonstration that local CBF increased insignificantly in relation to white matter but dramatically in relation to gray matter. The apparently limited vasodilatory capacity in white matter is discussed in the section on CBF, but this imbalance between glucose needs and glucose delivery may contribute to the propensity of neonatal cerebral white matter to hypoxic injury .
SITE | MECHANISM a |
---|---|
Plasma membrane | ↑ Ca 2+ influx through voltage-dependent Ca 2+ channels (cell depolarization) |
↑ Ca 2+ influx through agonist-dependent Ca 2+ channels (glutamate action at NMDA and immature AMPA receptors) b | |
Activation of phospholipase C and liberation of IP 3 (see below) (glutamate action at metabotropic receptor) | |
↓ Ca 2+ efflux through ATP-dependent uniport system (ATP depletion) | |
↓ Ca 2+ efflux through Na + -dependent antiport system (↓ extracellular Na + ) | |
Endoplasmic reticulum | ↑ Ca 2+ release to cytosol through effect of IP 3 (see above) |
↓ Ca 2+ uptake by ATP-dependent uniport system (ATP depletion) | |
Mitochondrion | ↑ Ca 2+ release to cytosol via Na + ,H + –dependent antiport system (↑ cytosolic Na + ,H + ) |
a The primary effect of ischemia to cause the indicated change in Ca 2+ homeostasis is shown in parentheses.
Mechanisms
The mechanisms for the biochemical effects relate to several factors ( Table 13.13 ). ATP levels are preserved initially at the expense of PCr. The initial fall in PCr, the principal storage form of high-energy phosphate in brain, relates primarily to the shift in the creatine phosphokinase reaction induced by the hydrogen ion (H + ) generated with lactate formation by anaerobic glycolysis ( Fig. 13.23 ). Later, the creatine phosphokinase reaction is driven by elevated concentrations of both ADP and H + . The initial acceleration of glycolysis and the glycogenolysis may relate to primarily a rise in cyclic AMP levels in the brain, demonstrated to be approximately threefold in the rat after only 30 seconds of nitrogen breathing. Cyclic AMP leads to activation of phosphorylase for glycogenolysis and of phosphofructokinase (and hexokinase) for glycolysis. Further activation of phosphofructokinase and hence glycolysis occurs as ATP levels fall and ADP and Pi levels rise. The fall in brain glucose occurs because the continued excessive utilization of glucose through anaerobic glycolysis, a most inefficient means of generating ATP, outstrips the capacity for glucose delivery from blood. Indeed, after 6 minutes, brain glucose levels had decreased by more than 70%, whereas blood glucose levels had increased by nearly 100% (see Fig. 13.3 ). Thus blood glucose level no longer reflected the brain glucose level, an observation of particular clinical relevance .
CALCIUM ACTION | DELETERIOUS EFFECT |
---|---|
Activate phospholipases | Phospholipid hydrolysis and membrane injury |
Generation of arachidonic acid and ultimately free radicalsby cyclooxygenase and lipoxygenase pathways | |
Activate proteases, disassembly of microtubules | Cytoskeletal disruption (caused by microtubular disruption and proteolysis of neurofilaments) |
Proteolysis of other cellular proteins | |
Activate nucleases | Nuclear injury |
Activate calcium-ATPase and other energy-dependent calcium extrusion mechanisms | Consumption of ATP at a time of deficient ATP |
Enter mitochondrion and uncouple oxidative phosphorylation | Decrease in ATP production |
Increase neurotransmitter release (e.g., glutamate, catecholamines) | Activation of glutamate receptors (e.g., calcium influx) |
Autooxidation of catecholamines with production of free radicals | |
Activate a protease for transformation of xanthine dehydrogenase to xanthine oxidase | Oxidation of hypoxanthine to xanthine and of xanthine to uric acid with production of free radicals |
Activate nitric oxide synthase | Generation of nitric oxide and ultimately peroxynitrite, with toxic effect on neurons |

The accumulation of lactate and the associated H + is worthy of additional emphasis because this accumulation initially is a beneficial adaptive response to oxygen deprivation, but later it can be a serious deleterious factor. Thus, initially, the tissue acidosis leads to the generation of ATP from PCr (because of the shift in the creatine phosphokinase reaction) and also to an increase in CBF (because of the local effect of elevated perivascular H + concentration on vascular smooth muscle). However, with the progression of lactate formation, severe tissue acidosis develops, and three deleterious effects ensue. The first is an impairment of vascular autoregulation and the potential for ischemic injury to the brain when cerebral perfusion pressure falls (e.g., secondary to the often associated myocardial injury). Second, phosphofructokinase activity is inhibited by low pH, and thus the brain’s remaining source of ATP (i.e., glycolysis) is eliminated. Finally, advanced tissue acidosis leads directly to cellular injury and, ultimately, necrosis. A correlation between brain lactate concentration and cellular injury has been demonstrated in the primate brain (see the next section).
Effects of Hypoxia-Ischemia on Carbohydrate and Energy Metabolism
Major Changes
Hypoxic-ischemic insults are accompanied by effects on carbohydrate and energy metabolism in the brain ( Table 13.14 ) that exhibit important similarities to those observed with hypoxemia. Certain differences occur with the addition of ischemia. In earlier years, the most frequently used models with perinatal animals included decapitation, severe hypotension, or occlusion of blood vessels supplying the cranium. a
a References .
The most widely used model in the past 20 years has involved the Vannucci adaptation of the Levine model of unilateral carotid artery ligation, followed by systemic hypoxemia for generally 1 to 3 hours, a procedure that results in hypoxic-ischemic neuronal and white matter injury. The combination of hypoxemia and ischemia (i.e., hypoxic-ischemic insult ) is most relevant to the situation in vivo in the human fetus and newborn. The effects of such an insult on carbohydrate and energy metabolism have been studied in detail in experimental models. bb References .
Sources |
Mitochondrial electron transport system |
Action of cyclooxygenase and lipoxygenase on arachidonic acid |
Action of xanthine oxidase on hypoxanthine and xanthine |
Autooxidation of catecholamines |
Infiltrating reactive microglia |
Action of nitric oxide synthase |
Endogenous defenses |
Major: superoxide dismutase (generates H 2 O 2 ), catalase (degrades H 2 O 2 ), and glutathione peroxidase (degrades H 2 O 2 ) |
Free radical scavengers: vitamin E (alpha-tocopherol), other sterols (21-aminosteroids), vitamin C (ascorbic acid), glutathione, other thiol compounds |
Major deleterious effects |
Peroxidation of PUFAs of membrane phospholipids (PUFAs especially abundant in brain membranes) |
Damage to DNA and to proteins containing unsaturated or sulfhydryl groups |
Activation of proapoptotic genes |
The biochemical features relative to carbohydrate and energy metabolism bear many similarities to those recorded previously for purely hypoxemic insults (see Table 13.14 ). In the most commonly used model, the hypoxic-ischemic insult is produced in the 7-day-old rat (approximately analogous to a preterm human newborn brain) by a combination of unilateral carotid occlusion and breathing of a low-oxygen (usually 8%) gas mixture. The importance of ischemia in the genesis of the brain injury in this model has been demonstrated by the findings that (1) carotid ligation alone does not lead to a decrease in CBF to the ipsilateral hemisphere, (2) the addition of the hypoxemia leads to marked disturbances in regional blood flow to the ipsilateral hemisphere, and (3) the topography of the injury to this hemisphere correlates closely with the topography of the decreases in regional CBF. Vannucci and colleagues defined the major biochemical changes most clearly. a
a References .
The initial biochemical changes are compatible with accelerated anaerobic glycolysis with lactate accumulation and glycogenolysis. Particular importance for an increased capacity for glucose uptake in the acceleration of glucose utilization has been shown by the demonstration of elevation in the levels of the glucose transporter proteins, GLUT1 (55 kDa) and GLUT3, for transport of glucose across the blood-brain barrier and the neuronal membrane, respectively, in the brain of hypoxic-ischemic 7-day-old rat pups in the first 4 hours after the insult. As with hypoxemia, a role for cyclic AMP in the induction of the glycolysis and glycogenolysis is suggested by marked rises (13-fold) in the levels of this mononucleotide in the first minutes after the onset of ischemia. Nevertheless, brain glucose concentrations fall more severely than with the anoxia of nitrogen breathing; after 2 minutes of ischemia, glucose had decreased markedly, whereas only a modest decrease occurred with nitrogen breathing after this time. Of course, this difference relates to the impairment of CBF and therefore glucose supply with ischemia. An additional difference between ischemia and hypoxemia is the more drastic increase in lactate and tissue acidosis with ischemia, because the circulation is interrupted. The more severe tissue acidosis obtains because the impaired cerebral circulation results in (1) diminished clearance of accumulated lactate and (2) diminished buffering of tissue carbon dioxide by the bicarbonate buffering system. The increased ratio of lactate to pyruvate in the cytosol is reflected in increased reduction (i.e., decrease) of the NAD + /NADH ratio. The latter ratio is more oxidized in the mitochondrion because of the limitation in cellular substrate (glucose) supply. (This important limiting role of brain glucose is discussed in more detail later concerning brain carbohydrate status and hypoxic-ischemic injury.) Perhaps most important, high-energy phosphate levels begin to decline within minutes, with the reservoir form, PCr, falling first ( Fig. 13.24 ). Histological evidence of brain injury becomes apparent after approximately 90 minutes.
The particular importance of ischemia in the genesis of the deleterious effects of hypoxic-ischemic insults was also shown in the fetal lamb and neonatal piglet. In both animal models, marked hypoxemia did not result in brain injury unless hypotension supervened . In the piglet, hypotension appeared to be a particular consequence of cardiac dysfunction, and the latter was especially correlated with severe systemic acidosis. In the fetal lamb, pronounced decreases in brain glucose and in high-energy phosphate levels, accompanied by an increase in lactate levels to as high as 16 to 24 mM, were the principal biochemical effects on carbohydrate and energy metabolism. These effects were particularly pronounced in cerebral white matter ( Table 13.15 ). This regional predilection may be relevant to the propensity of white matter to exhibit injury with hypotension in the premature newborn (see Chapter 14 , Chapter 15 , Chapter 16 ).
TYPE | FUNCTION |
---|---|
Ionotropic | |
NMDA | Ca 2+ entry, Na + entry |
AMPA | Na + entry, Ca 2+ entry ( immature neurons ) b |
Kainate | Na + entry |
Metabotropic | |
Ibotenate | Phosphoinositide hydrolysis; protein kinase C activation; Ca 2+ mobilization from endoplasmic reticulum; multiple downstream effects |
a The receptors are named according to the glutamate analogue most potent in activation of the individual receptor.
b In immature neurons AMPA receptors lack the GluR2 subunit, the subunit which renders the receptor Ca 2+ impermeable, thus resulting in Ca 2+ -permeable receptors.
Secondary Energy Failure
The temporal aspects of the changes in glucose and energy metabolism after hypoxic-ischemic insult in the living animal have been identified best by studies of the neonatal piglet with phosphorus and proton MR spectroscopy and have defined a delayed, secondary energy failure. Thus, immediately after the insult, as expected, a marked increase in cerebral lactate levels and a marked decrease in high-energy phosphate levels were documented (i.e., primary energy failure). High-energy phosphate levels recovered to baseline levels in 2 to 3 hours ( Fig. 13.25 ); lactate levels improved but did not recover completely. A second decline in high-energy phosphate levels then occurred in the next 24 hours and was especially pronounced at 48 hours (see Fig. 13.25 ). This secondary energy failure and the earlier rise in cerebral lactate levels have been documented in the human term newborn subjected to apparent hypoxic-ischemic insult in the context of perinatal asphyxia (see Chapter 18 , Chapter 19 , Chapter 20 ). The onset of the secondary decline in high-energy phosphates varies according to the species and nature of the insult, but in general the onset is clear by 8 to 16 hours and reaches a nadir at 24 to 48 hours. A major question has been whether the secondary energy decline causes or accentuates brain injury or whether the decline is a consequence of the injury .

A particularly informative study of the neonatal rat (unilateral carotid ligation and hypoxemia on postnatal day 7) confirmed the occurrence of secondary energy failure, with onset at approximately 18 to 24 hours and a nadir at 48 hours. However, immunocytochemical studies showed that the loss of neuronal proteins became apparent at 6 hours and was very pronounced at 18 hours (i.e., before the onset of the secondary energy failure). The temporal characteristics and the additional finding of a loss of total creatine and adenine nucleotides supported the conclusion that the secondary energy depletion is a consequence rather than a cause of cellular destruction. As discussed earlier, in the hours after the hypoxic-ischemic insult, a cascade of events, including accumulation of excitotoxic amino acids, cytosolic Ca 2+ , activation of phospholipases, generation of free radicals, and a series of related metabolic events, develops and leads to cell death. The crucial mitochondrial disturbance that precipitates this cascade of deleterious events is responsible for the primary energy failure and persists into the period after the termination of the insult despite initial recovery of high-energy phosphates (see later discussion). The particular vulnerability of the mitochondrion during and after ischemia is supported by biochemical and morphological data. a
a References .
Investigators have suggested that the secondary energy failure initiates the cascade of events just noted. However, the work just described appears to favor the notion that the secondary energy depletion is a consequence of the cascade of events and the resulting cell death.Effects of Asphyxia on Carbohydrate and Energy Metabolism
Asphyxia, rather than hypoxemia or ischemia or both, is the most common clinical insult in the perinatal period that results in the brain injury under discussion. Although hypoxemia and ischemia usually occur concurrently or in sequence with perinatal asphyxia, certain additional metabolic effects, particularly hypercapnia, are prominent. Most experimental studies of perinatal asphyxia have involved lambs and monkeys and have been concerned with changes in CBF and with the neuropathology (see later sections on CBF and Chapters 14 , 15 , 18 , and 19 ). Some work has provided useful information regarding the biochemical (as well as the physiological) effects in the brain with neonatal asphyxia and is reviewed next.
Major Changes
Striking changes in biochemical, cardiovascular, cerebrovascular, and electrophysiological parameters were observed in neonatal dogs subjected to ventilatory standstill after paralysis with succinylcholine or curare. Survival occurred in all animals after 10 minutes of asphyxia, in two-thirds after 15 minutes of asphyxia, but in only one-fourth after 20 minutes of asphyxia. Changes in arterial blood gas levels and acid-base status were dramatic. Thus, after minutes of respiratory arrest, PaO 2 had fallen to 4 mm Hg, partial pressure of carbon dioxide (PaCO 2 ) had risen to 51 mm Hg (from control value of 35), and pH had fallen to 7.18 (from control value of 7.38). After 10 minutes, PaCO 2 was 100 mm Hg, and pH was 6.79. Cardiovascular effects were also marked ( Fig. 13.26 ); MABP declined gradually to a low of 10 mm Hg after 14 minutes, and bradycardia was marked after only 4 minutes. Cerebral perfusion , assessed qualitatively by carbon black infusion, overall appeared to decline pari passu with MABP, although diminutions were greatest in cerebral cortex and least in brain stem. This more severe affection of cerebral flow has been reproduced in other neonatal models of asphyxia (see later discussion). The EEG demonstrated rapid deterioration (see Fig. 13.26 ); between 1 and 2 minutes after the onset of asphyxia, a distinct reduction in the amplitude and frequency occurred, and by
minutes, the EEG was isoelectric. The occurrence of the isoelectric EEG did not correlate with any marked change in cerebral perfusion or with any measurable change in brain lactate or ATP levels. In the asphyxiated fetal sheep, this suppression of EEG, initially an energy-conserving protective effect, is mediated by adenosine, an inhibitory neurotransmitter.

Biochemical effects were qualitatively similar to those observed with hypoxemia or ischemia or both ( Figs. 13.27 and 13.28 ). Thus the brain glucose level declined rapidly (despite normal blood glucose level), lactate concentration rose (after a -minute delay), and PCr concentration decreased markedly (to values ≈20% of control within 5 minutes). However, ATP levels were maintained for 6 minutes of asphyxia but then declined by 10 minutes. The changes in high-energy phosphates have been documented in the living animal by MR spectroscopy. Thus, after 5 minutes of asphyxia, in which electrocerebral silence occurred after 3 minutes, a 40% decrease in the PCr/Pi ratio and a 30% decrease in the ATP/Pi ratio occurred. Despite these changes, on reinstitution of ventilatory support, cerebral metabolism returned to normal within 20 to 30 minutes. However, studies in neonatal piglets showed that during a similar recovery period after an even less severe asphyxial insult (2 to 3 minutes), evidence for lipid peroxidation and altered membrane function (depressed Na + /K + -ATPase activity) was demonstrable. Production of intrauterine asphyxia by impairment of placental blood flow also decreases cerebral high-energy phosphate levels, as measured by MR spectroscopy in the living animal.


Additional Effects of Asphyxia (vs. Solely Hypoxemia or Ischemia or Both)
At least four major factors are added to the constellation of biochemical features controlling the outcome of asphyxia, with its attendant increase in PaCO 2 . The first three of these factors appear to be beneficial, at least initially, and the fourth of these appears deleterious. First , the hypercapnia acts to maintain or even augment CBF through an increase in the perivascular H + concentration in the brain, which may be beneficial early in asphyxia. Second , the hypercapnia may be associated with a diminution in cerebral metabolic rate. Moderate hypercapnia has been shown to cause a diminution in cerebral metabolic rate in the adult rat brain, adult monkey brain, and developing rat brain. Third , an increase in PaCO 2 leads to acidemia, which is accompanied by a shift in the oxygen-hemoglobin dissociation curve such that the affinity of hemoglobin for oxygen is decreased. The result is an increase in the delivery of oxygen to cells. The operation of one or more of these three factors could underlie the protective effect of moderate hypercapnia in immature rats subjected to hypoxia-ischemia. The fourth important factor relative to hypercapnia and the outcome with asphyxia may be deleterious; intracellular pH falls more drastically for a given amount of lactate formed when the effect of elevated PCO 2 is added by asphyxia. Thus extreme acidosis and consequent tissue injury could result. Future studies directed at defining the relative roles of these four factors in the genesis of the biochemical and physiological derangements associated with asphyxia in the perinatal animal will be of great interest.
Influence of Carbohydrate Status on Hypoxic-Ischemic Brain Injury
Deleterious Role of Low Brain Glucose in Perinatal Animals
A series of older studies with immature animals suggests a beneficial effect of prior administration of glucose and a deleterious effect of hypoglycemia on the survival response to anoxic insult (i.e., nitrogen breathing). The effects of glucose appeared to be exerted on the central nervous system rather than on the heart, because the time to last gasp was altered before cardiac function. This observation is compatible with data indicating the particular resistance of the immature heart to combined hypoxia and hypoglycemia, presumably because of rich carbohydrate stores and high glycogenolytic and glycolytic capacities. Later work on the survival and neuropathological response to hypoxia and ischemia of neonatal animals also has demonstrated a beneficial effect of pretreatment with glucose and a deleterious effect of hypoglycemia ( Fig. 13.29 ). One study of 185 term human infants who had sustained apparent intrapartum asphyxia (markedly low cord pH) showed a deleterious effect of initial hypoglycemia on neurological outcome. Thus, of infants who had initial blood glucose values lower than 40 mg/dL, 56% had an abnormal neurological outcome, versus only 16% among those with initial blood glucose values higher than 40 mg/dL. This apparent deleterious effect of hypoglycemia was also documented in a study focused on the neuroimaging correlates of hypoglycemia in the term-born infant that revealed patterns of brain injury similar to those of hypoxic-ischemic injury. Importantly, in this study the majority of infants had low initial Apgar scores with or without acidemia, suggesting that the hypoglycemia was complicating an underlying asphyxial cerebral insult.

Importance of Endogenous Brain Glucose Reserves.
The biochemical mechanisms for the relation between carbohydrate status and resistance to hypoxic-ischemic insult relate to glycolytic capacity. Thus, with hypoxic-ischemic states, the replenishment of brain high-energy phosphate levels depends on anaerobic glycolysis. Because of the 19-fold reduction in ATP production per molecule of glucose when the brain is forced to oxidize glucose anaerobically, the glycolytic rate must be enhanced greatly. The adaptive mechanisms that come into play for this purpose are summarized in the previous sections. The greatly enhanced glycolytic rate leads to a decline of brain glucose levels. a
a References .
If this decline is prevented (e.g., by prior administration of glucose), the glycolytic rate and hence ATP production are increased, and the biochemical and clinical outcome for animals rendered hypoxic or partially ischemic is improved considerably. bb References .
Indeed, the careful studies of Vannucci et al. indicated that the major factor accounting for the difference in outcome between normoglycemic and hypoglycemic animals rendered hypoxic is the amount of endogenous brain glucose reserves at the time of the insult. In hypoglycemic animals, a 10- to 20-fold reduction in endogenous brain glucose resulted and correlated best with the impaired glycolytic rate and the decline in high-energy phosphate levels in brain with nitrogen breathing. Brain glycogen levels seemed less important. Thus the capacity for surviving hypoxemia was reduced fivefold in hypoglycemic animals at a time (i.e., 60 minutes after insulin injection) when the brain glycogen level was reduced by only 20%, but brain glucose level was reduced by more than 10-fold ( Fig. 13.30 ). Similarly, reversal of the vulnerability correlated with a rapid normalization of brain glucose levels but no significant change in brain glycogen levels.
Summary.
Taken together, these data on immature animals (principally rodents) indicate that carbohydrate status plays an important role in determining the biochemical and clinical responses to hypoxemic and ischemic insults. Hypoglycemia is deleterious, and pretreatment with glucose is beneficial. The mechanism of the effect appears to relate to changes in endogenous, readily mobilized brain glucose reserves, which lead to the enhanced glycolytic rate required to slow the decline of, or even maintain the levels of, high-energy phosphate in the brain.
Deleterious Role of Abundant Brain Glucose in Adult Animals
A potentially deleterious role for abundant brain glucose in the clinical, pathological, and biochemical responses to hypoxemia and ischemia was suggested initially by studies with juvenile rhesus monkeys. In a series of experiments with animals routinely food deprived for 12 to 24 hours before subjection to circulatory arrest, investigators showed that a period of circulatory arrest as long as 14 minutes was compatible with apparently good neurological recovery and “minimal” neuropathological abnormalities, restricted principally to brain stem nuclei, hippocampus, and Purkinje cells. However, animals that were administered an infusion of 1.5 to 3 g/kg of glucose (5% dextrose in saline) that terminated 10 minutes before the 14-minute period of circulatory arrest did very poorly. The clinical course was characterized by seizures, hypertonia, and ultimately decerebrate rigidity, evolving over hours. On sacrifice, in glucose-pretreated monkeys, in contrast to the food-deprived monkeys, the glucose exhibited “changes indicative of widespread injury to tissue…and diffuse cytologic injury” with widespread involvement of the cerebral cortex. In a subsequent study, glucose was administered as a 50% solution in a dose of 2.5 to 5 g/kg 15 minutes before circulatory arrest, and similar clinical and neuropathological consequences were observed.
Importance of Severe Lactic Acidosis in Brain.
The biochemical mechanism for the deleterious effect of pretreatment with glucose in the previously mentioned juvenile monkeys may relate to the greater accumulation of lactic acid in the glucose-pretreated than in the food-deprived (control) monkeys ( Table 13.16 ). ATP levels declined approximately 10-fold in food-deprived animals subjected to circulatory arrest, and only a minimal difference in the magnitude of that decline was observed in animals pretreated with glucose. However, whereas lactate levels increased approximately fourfold in the food-deprived animals subjected to circulatory arrest, the levels increased more than 10-fold in those pretreated with glucose. The greater increases in brain lactate levels in the glucose-pretreated animals presumably reflected higher endogenous brain glucose reserves and, as a consequence, enhanced lactate production by anaerobic glycolysis. These experiments and related observations with animals rendered severely hypoxemic led Myers and Yamaguchi to suggest that the accumulation of brain lactate to concentrations of approximately 20 mmol/kg or greater leads to tissue destruction and brain edema. This approximate threshold level is supported by the observations that the accumulation of lactate to higher than this level occurs in thebrain of monkeys rendered ischemic in those regions that have been shown to be particularly vulnerable to neuronal injury.
SITE | EFFECT ON EXCITATION | |
---|---|---|
INCREASE | DECREASE | |
Presynaptic (glutamate release) | Calcium | Magnesium |
Theophylline | Adenosine | |
Receptor | Glutamate | APV/CPP |
Glycine | Kynurenate | |
Channel | MK-801/ketamine/dextromethorphan | |
Magnesium/memantine |
Considerable support for the concept of a deleterious effect of abundant glucose and resulting lactic acidosis in brain in the pathogenesis of hypoxic-ischemic brain injury in the adult was provided by further studies in a variety of experimental models in mature animals . A threshold value of lactate of approximately 20 mmol/kg, above which major tissue injury occurs, can be suggested from the data. The apparent mechanism for the principal injury from these high levels of lactate is injury to endothelial cells, and perhaps also to perivascular astrocytes, with resulting disturbance of cerebral perfusion. Direct neuronal injury is likely, but widespread, secondary ischemic injury appears to develop primarily because of the vascular changes.
Beneficial(?) Role of Abundant Brain Glucose in Perinatal Animals
In contrast to the deleterious role for glucose in hypoxic-ischemic injury in adult animals, considerable data in the immature rat suggest a beneficial role for abundant glucose administered primarily during or at the termination of the insult. a
a References .
Hattori and Wasterlain, using a model of bilateral carotid occlusion and ventilation with 8% oxygen for 1 hour, showed marked reduction of neuropathological injury in animals treated with supplemental glucose at the termination of the hypoxic breathing ( Fig. 13.31 ). Supplementation 1 hour after termination of the hypoxia had no beneficial effect. In a neonatal lamb model of asphyxia, glucose supplementation prevented the prolonged postasphyxial impairment in cerebral oxygen consumption observed in control (or hypoglycemic) animals ( Fig. 13.32 ). Moreover, neonatal rats breathing 8% oxygen survived twice as long when they were treated with 50% glucose; 50% survival was approximately 4 hours in saline-treated animals versus 8 hours in glucose-treated animals.

The mechanism for any beneficial effect of glucose in these perinatal models of hypoxia-ischemia is not conclusively known but probably relates to preservation of mitochondrial energy production. Thus Yager et al. showed that glucose supply becomes limiting in hypoxia-ischemia (unilateral carotid occlusion and 8% oxygen breathing) in the neonatal rat, a conclusion based on the relatively oxidized state of mitochondrial NAD + /NADH. Brain glucose levels clearly increase after glucose supplementation, in several models of hypoxia-ischemia. With the model of carotid occlusion and 8% oxygen breathing, brain levels of high-energy phosphates were clearly higher in glucose-treated versus saline-treated animals ( Table 13.17 ). In addition, brain lactate levels in the hypoxic-ischemic neonatal rats were considerably higher in the glucose-treated animals. Indeed, after 2 hours of hyperglycemia, brain lactate levels reached 25.5 mmol/kg. However, no evidence for tissue injury caused by the elevated brain lactate levels was reported, in contrast to the negative effect in the more mature brain. Moreover, in other perinatal models (in the near-term fetal sheep, the newborn lamb, and the newborn dog), brain lactate levels did not rise to such levels with hypoxia-ischemia or asphyxia. Increase in brain lactate levels in neonatal brain relative to adult brain is limited by the lower capacity for glucose uptake by the glucose transporter proteins, especially GLUT1 (55 kDa), and by lower hexokinase activity, the rate-limiting enzyme for glucose utilization. a
a References .
Indeed, the possibility should be considered that any increase in lactate that may occur in the glucose-treated animal is used for energy production (by oxidation to pyruvate and entrance into the tricarboxylic acid cycle), because lactate is a preferred fuel in the neonatal brain. Moreover, lactate is transported rapidly across the blood-brain barrier in the immature animal, and at least in the rat, brain pH normalizes by 10 minutes of recovery and tissue lactate levels normalize by 4 hours, unlike the prolonged tissue acidosis that occurs in adult rats subjected to hypoxia-ischemia and glucose treatment. Indeed the combination of rapid utilization of lactate by brain and rapid efflux from brain may explain the lack of serious tissue injury by levels of lactate that lead to injury in the adult brain.In developing neurons in cell culture, excitatory synaptic activity is necessary for hypoxia to lead to neuronal death. |
Nonspecific blockade of this synaptic activity prevents hypoxic neuronal death in culture. |
Specific glutamate receptor channel blockers also prevent hypoxic neuronal death in culture and in brain slices. |
Glutamate accumulates extracellularly in vivo with hypoxic-ischemic insult. |
Topography of hypoxic-ischemic neuronal death in vivo is similar to the topography of glutamate synapses. |
Increased vulnerability of certain brain structures to hypoxic-ischemic injury during early development correlates, in those structures, with transiently increased concentration of glutamate receptors, which also have molecular characteristics associated with increased calcium influx. |
Ontogeny of hypoxic-ischemic neuronal death in vivo is similar to the ontogeny of glutamate-induced neuronal death. |
Delayed neuronal death after glutamate exposure in cell culture has a correlate in delayed neuronal death after hypoxia-ischemia in vivo, and both can be prevented by specific glutamate receptor channel blockers, some administered after termination of the insult. |
Enthusiasm for supplementation with glucose during or after hypoxic-ischemic insults in the immature brain must be tempered by the results of four other studies of young animals. Thus, in a model of focal ischemia in the 7-day-old rat, glucose administration after hypoxia-ischemia led to more severe neuronal injury (although no increase in infarct size) than did saline administration. Moreover, in a model of global hypoxia-ischemia in 1- to 3-day-old piglets, glucose administration during the insult led to accentuated neuronal injury. Glucose administration after the insult did not ameliorate the injury, as such therapy accomplished in the immature rat (see earlier). Finally, studies of fetal sheep at 80 days of gestation subjected to bilateral carotid occlusion show no effect on the degree of brain injury of prolonged moderate hyperglycemia before ischemia and during reperfusion, but a clear detrimental effect when an acute and marked increase in plasma glucose concentration (approximately sevenfold greater than control values) was added to the moderate hyperglycemia just before ischemia. Brain lactate levels were not measured. The reasons for the differences in results obtained in the several perinatal models are unclear but may relate to methodological differences.
Summary.
Current experimental data allow several tentative conclusions to be made about the effects of glucose administration with perinatal hypoxic-ischemic insults ( Table 13.18 ). On balance, the findings favor maintenance of blood glucose concentrations in the normal range in infants who have sustained hypoxic-ischemic insults or who are at risk for sustaining such insults.
Microglial activation occurs promptly and briskly after hypoxia-ischemia. |
Microglial cells release neurotoxic products (e.g., cytokines and reactive oxygen and nitrogen species). |
Cytokines (especially IL-1beta and TNF-alpha) increase promptly in brain after hypoxia-ischemia, and IL-1 receptor antagonists ameliorate hypoxic-ischemic brain injury when they are administered either before or at the termination of hypoxia-ischemia. |
Antimicroglial agents (e.g., minocycline) are neuroprotective in several experimental paradigms of perinatal hypoxia-ischemia. |
Inflammatory mechanisms provoked by prior exposure to molecular products of infection (e.g., lipopolysaccharide) potentiate subthreshold hypoxic-ischemic insults to lead to severe brain injury. |
Influence of Maturation on Glucose and Energy Metabolism With Hypoxia-Ischemia
The influence of the maturational state of the brain on the severity and topography of the brain injury caused by hypoxia-ischemia is complex. It is now clear that the long-held general notion that the perinatal brain is more resistant than the adult brain is too simplistic. Evidence indicates that cerebral glucose and energy metabolism are more resistant to perturbation by hypoxia-ischemia in the immature than in the adult brain. a
a References .
Some of the mechanisms underlying the resistance of energy metabolism in the immature brain are summarized in Table 13.19 . However, neuropathological studies indicate that many critical neuronal groups are more vulnerable to hypoxic-ischemic injury in the immature animal. This vulnerability of immature neurons relates particularly to enhanced density and function of excitatory amino acid receptors and enhanced vulnerability to attack by reactive oxygen species (ROS) and reactive nitrogen species (RNS), as discussed later. A particular vulnerability of immature oligodendrocytes to hypoxic-ischemic, excitatory amino acid, and free radical injury is discussed later but also is relevant in this context. The various influences of maturation on the regional aspects of hypoxic-ischemic brain injury and on the responses to interventions are highlighted in appropriate subsequent sections of this chapter.Decrease of energy depletion |
Hypothermia |
Glucose |
Sedation |
Hypercapnia (mild) |
Inhibition of glutamate release |
Hypothermia |
Calcium channel blockers |
Magnesium |
Adenosine or adenosine agonists |
Free radical scavengers |
Anticonvulsants such as lamotrigine, levitaretam |
Phenytoin |
Cannabinoids |
Amelioration of impairment in glutamate uptake |
Hypothermia |
Blockade of glutamate receptors |
NMDA receptor antagonists (MK-801, xenon, memantine, magnesium, ketamine, dextrorphan) |
Non-NMDA receptor antagonists (NBQX, CNQX, topiramate) |
Blockade of free radical generation |
Hypothermia |
Inhibitors of cyclooxygenases (e.g., indomethacin) |
Inhibitors of lipoxygenases (e.g., AA-861) |
Nitric oxide synthase inhibitors (e.g., nitroarginine derivatives, 2-iminobiotin) |
Allopurinol |
Iron chelators |
Fructose-1,6-biphosphate |
Removal of free radicals |
Free radical scavengers (vitamin E and analogues, edaravone, N -acetylcysteine) |
Antioxidant enzyme mimetics |
Blockade of downstream effects |
Hypothermia |
Erythropoietin |
Growth factors (insulin-like growth factor-I, brain-derived neurotrophic factor, nerve growth factor) |
Caspase inhibitors |
Inhibition of inflammatory effects |
Hypothermia |
Minocycline |
Anti–Toll-like receptor agents |
Interleukin-1 receptor antagonists |
Platelet-activating factor antagonists |
Neutropenia |
Birth as an Additive or Potentiating Factor in Hypoxic Injury
Perinatal hypoxic-ischemic injury occurs in the setting of a profound alteration of biochemical and physiological homeostasis (i.e., the process of birth). Transient hypoxemia and hypercapnia, variable in severity and duration, are consistent occurrences. Transient disturbances in CBF may also occur. It is appropriate to ask whether the biochemical state of the brain is affected by the major systemic alterations that take place at birth.
Careful studies of the perinatal rat brain indicate that spontaneous vaginal delivery is associated with the signs of hypoxemic or ischemic insult to the brain . Alterations in glycogen, certain glycolytic intermediates, and high-energy compounds after spontaneous vaginal delivery are shown in Table 13.20 . Evidence for glycogenolysis, enhanced lactate production, PCr conversion to ATP, and a decline in ATP concentrations is apparent in the first minute after birth. Simultaneous elevation of the concentrations of glucose-6-phosphate and glucose-1-phosphate and decline of the concentration of glycogen are consistent with the occurrence of glycogenolysis. The elevation of lactate level and of the lactate/pyruvate ratio indicates enhanced anaerobic glycolysis. The sharp decline of PCr is not adequate in the first minute to preserve ATP concentrations, and in fact the small persisting deficit in ATP levels 10 minutes after birth is statistically significant. Not shown, but accompanying these changes, is a decline in brain glucose concentrations relative to blood glucose, a finding reflecting further the enhanced glycolysis. By 1 hour after delivery, high-energy phosphate concentrations were no longer depressed, and the ratio of lactate to pyruvate was considerably improved. The latter was normal by 8 hours after delivery. Determinations of identical parameters after cesarean section showed very transient and much smaller changes; indeed, no significant change in ATP levels was observed at any time after delivery by cesarean section.
Principal features |
Timing: commence before delayed energy failure and excitatory features such as seizures (i.e., ≈6 h) |
Degree: decrease body temperature 3–4°C |
Duration: ≈72 h |
Mechanisms of benefit |
Decrease energy consumption |
Decrease accumulation of glutamate |
Decrease synthesis of reactive oxygen and nitrogen species |
Block downstream molecular cascade to apoptosis |
Inhibit inflammatory mechanisms |
Synergistic neuroprotective combinations |
Hypothermia and xenon |
Hypothermia and topiramate |
Hypothermia and N -acetylcysteine |
Hypothermia and caspase inhibitor |
These data indicate that the process of birth through the vaginal route in a neonatal animal model is associated with the biochemical signs of hypoxic insult to brain. The influence of this phenomenon on the impact of hypoxic-ischemic insults occurring before or immediately after birth is not known. An intuitive conclusion would be that the insult at birth may be additive. However, it is possible that the insult at birth may play a protective role in relation to a subsequent insult. Thus a degree of protection to hypoxic-ischemic neuronal injury has been shown in the immature rat by prior exposure to hypoxia. This hypoxic preconditioning appears to be related to induction of multiple genes, particularly the transcription factor hypoxia-inducing-factor 1 (HIF1) and its target genes, that blunt the adverse effects of hypoxia-ischemia by induction of vasodilation, glucose transport, glycolysis, and antiexcitotoxic, antioxidant, antiapoptotic, and other mechanisms.
Biochemical Mechanisms of Cellular Injury in Hypoxic-Ischemic Cerebral Injury: Beyond Glucose and Energy Metabolism
The principal biochemical mechanisms of cell death with hypoxia-ischemia and asphyxia are presumably very similar, if not identical, and are initiated by oxygen (and glucose) deprivation and an impairment in energy supplies. Because all the principal, currently considered mechanisms for cell death with oxygen deprivation at least begin with the disturbances of brain glucose and energy metabolism, it is appropriate to synthesize current concepts concerning the mechanisms for cell death immediately after the preceding sections, which emphasize these disturbances. Nevertheless, it is now clear that the mechanisms of cell death with oxygen deprivation are not simply the result of energy failure and, indeed, extend beyond glucose and energy metabolism. An enormous amount of literature attests to the complexity of the mechanisms. In the following section, we attempt to synthesize the essential data and to isolate the most critical mechanisms. The emphasis is on mechanisms of neuronal death . Although mechanisms of white matter injury, especially oligodendroglial death , bear many similarities, sufficient differences warrant separate consideration in the next major section .
As discussed in the next section, the cascade of deleterious events that lead to cell death after insults that result in oxygen deprivation and energy failure appears to occur primarily following termination of the insult . Careful studies in animal models and in human patients provide strong support for this notion. The phenomena are initiated particularly by energy depletion, accumulation of extracellular excitatory amino acids (particularly glutamate), increase in cytosolic calcium, and generation of free radicals. The importance of this “delayed” death of the brain in the hours after the termination of the insult is related in largest part to the possibility that intervention during the postinsult period could be beneficial. The temporal evolution to cell death is shown in Figs. 13.6 and 13.33 .

Role of Accumulation of Cytosolic Calcium
A large body of information indicates a major role for accumulation of cytosolic Ca 2+ during and after hypoxia-ischemia in the mediation of cell death. In perinatal models of hypoxia-ischemia, increased Ca 2+ uptake into the insulted brain regions, close correlation within brain regions between increased uptake of Ca 2+ and subsequent neuronal injury, and some protection from subsequent brain injury by pretreatment with voltage-dependent Ca 2+ channel antagonists have been documented. a
a References .
Moreover, because a major mechanism of Ca 2+ influx into the cytosol is through the N -methyl- d -aspartate (NMDA) and alpha-amino-3-hydroxy-5-methyl-4-isoxazolepropionic acid (AMPA) types of glutamate receptors, the protection from brain injury afforded by antagonists of these receptors (see later discussion) may be mediated primarily by decreasing the accumulation of cytosolic Ca 2+ .Mechanisms.
The mechanisms by which increased cytosolic Ca 2+ leads to cell death are multiple but are best discussed in the context of normal Ca 2+ homeostasis ( Fig. 13.34 ). The cellular mechanisms for maintenance of low cytosolic Ca 2+ concentrations (10 −7 M) relative to high extracellular Ca 2+ concentrations (10 −3 M) are located in the plasma membrane (voltage-dependent channels; three agonist-dependent, that is, glutamate-dependent, channels: the NMDA, AMPA [immature, i.e., lacking GluR2 subunit], and metabotropic receptor-activated channels [see later discussion]; an ATP-dependent uniport system, and an Na + -dependent antiport system), the endoplasmic reticulum (an ATP-dependent import system and a release mechanism activated by inositol triphosphate), and the mitochondrion (a voltage-dependent channel and an Na + /H + -dependent antiport system; see Fig. 13.34 ). The mechanisms for the increased cytosolic Ca 2+ in neurons subjected to hypoxia-ischemia, and the metabolic and ionic changes caused by ischemia that underlie these mechanisms, are summarized in Table 13.21 . The central roles for ATP depletion, membrane depolarization, and voltage-dependent and glutamate-activated Ca 2+ channels are apparent .

Upstream initiating mechanisms |
Antiexcitotoxic (attenuation of glutamate release) |
Antioxidant effects |
Inhibition of nitric oxide production |
Preservation of autoregulation (?) |
Downstream mechanisms |
Antiapoptotic effects |
Survival promoting effects |
Stimulation of angiogenesis |
Stimulation of neurogenesis |
Anti-inflammatory effects (?) |
The deleterious effects of increased cytosolic Ca 2+ are multiple and affect the cell in a variety of ways ( Table 13.22 ). b
b References .
These effects include degradation of cellular lipids by activation of phospholipases, of cellular proteins (especially cytoskeletal elements) by activation of proteases, and of cellular DNA by activation of nucleases, as well as crucial indirect mechanisms of destruction mediated by generation of free radicals and nitric oxide (NO; Fig. 13.35 ). The utilization of ATP by ATP-dependent Ca 2+ -transport systems, attempting to correct the cytosolic Ca 2+ accumulation, and the Ca 2+ -mediated uncoupling of oxidative phosphorylation serve to perpetuate the process (see Fig. 13.35 ).AMPA receptors (GluR2-deficient) are concentrated on preoligodendrocyte somata, and NMDA receptors are concentrated on preoligodendrocyte processes. |
These receptors are overexpressed in cerebral white matter during the peak period of vulnerability to hypoxic-ischemic white matter injury. |
Both GluR2-deficient AMPA receptors and NMDA receptors are permeable to calcium. |
Ischemia leads to calcium influx through activation of these receptors with either cell death (AMPA activation) or loss of processes (NMDA activation), or both. |
Both receptors can be blocked by specific antagonists and lead to protection. |

Role of Free Radicals: Reactive Oxygen and Nitrogen Species
Free Radicals.
The crucial role of free radicals, generated in considerable part by Ca 2+ -activated processes just described, in the mediation of cell death with hypoxia-ischemia has been established by the study of a variety of models in vivo, in culture, and in vitro (see later). The emphasis in these studies has been neuronal injury. Discussion of the role of free radicals in oligodendroglial injury is contained in a later section. Free radicals are highly reactive compounds with an uneven number of electrons in the outermost orbital. These compounds can react with certain normal cellular components (e.g., unsaturated fatty acids of membrane lipids) and can generate a new free radical and thereby a chain reaction, which results in irreversible biochemical injury (e.g., peroxidation of the unsaturated fatty acids, membrane injury, and cell necrosis). With less intense insults, free radicals can lead to apoptotic cell death by activation of specific death genes. Free radicals in mammalian cells exist primarily as ROS (e.g., superoxide anion, hydrogen peroxide, hydroxyl radical) or as RNS (e.g., peroxynitrite [ONOO − ]; see later discussion). When these reactive species are present in excess, the cell is said to be subjected to oxidative stress or nitrative stress, respectively. Although studies have generally focused on ROS or RNS separately, these species usually exist together (e.g., under conditions of hypoxia-ischemia-reperfusion). I discuss the investigations of ROS and RNS in separate sections later.
The principal sources of free radicals with hypoxia-ischemia, the endogenous defenses against such radicals, and their major deleterious effects are summarized in Table 13.23 . Of the sources of free radicals with hypoxia-ischemia, the electron transport system is important when oxygen deprivation prevents the complete passage of electrons to cytochrome c oxidase. Free radicals, specifically superoxide anion, are then generated proximal to this terminal enzyme in the electron transport system. The next four sources are directly or indirectly related to cytosolic Ca 2+ (see Fig. 13.35 and Table 13.22 ). Arachidonic acid is generated by Ca 2+ -activated phospholipase A 2 , xanthine oxidase is activated by Ca 2+ , catecholamine release is stimulated by an increase in cytosolic Ca 2+ , and NO synthase (NOS; see next section) is activated by Ca 2+ . a
a References .
Finally, data indicate that early reactive cells at the site of initial insult, especially microglia , are potent sources of free radicals (see later discussion).LOCAL CHEMICAL FACTOR | CHANGE IN BRAIN EXTRACELLULAR FLUID | EFFECT ON CEREBRAL VESSELS | ||
---|---|---|---|---|
HYPOXIA | CORTICAL ACTIVITY b | INCREASE | DECREASE | |
Hydrogen ion | Increase | Increase | Dilate | Constrict |
Potassium ion | Increase | Increase | Dilate | Constrict |
Adenosine | Increase | Increase | Dilate | Constrict |
Prostaglandins | Increase | Increase | Dilate | Constrict |
Osmolarity | ? | ? | Dilate | Constrict |
Calcium ion | Decrease | Decrease | Constrict | Dilate |
Reactive Oxygen Species.
An important role for ROS in perinatal models of fetal and neonatal hypoxemic, ischemic, hypoxic-ischemic, and asphyxial insults now seems established. b
b References .
After the insults, generation of ROS or elevations of compounds known to lead to generation of ROS have been found. Moreover, studies principally of asphyxia in the newborn lamb and in the neonatal piglet and of hypoxia-ischemia (carotid ligation and low oxygen breathing) in the immature (7-day-old) rat have shown elevations of free radicals, deleterious effects of free radical attack (e.g., evidence for lipid peroxidation), or a neuroprotective effect of treatment (pretreatment, treatment during the insult, or after termination of the insult) with free radical scavengers (e.g., vitamin E analogues, edaravone, or drugs that inhibit free radical formation, such as allopurinol, oxypurinol, indomethacin, superoxide dismutase [SOD], catalase, and iron chelators). cc References .
The importance of the reperfusion period, rather than the time of the hypoxic-ischemic insult per se, in the generation of the ROS also has been emphasized ( Fig. 13.36 ).
The major endogenous antioxidant defense system is illustrated in Fig. 13.37 . Thus the most commonly generated initial oxygen free radical, the superoxide anion, is converted to hydrogen peroxide by the enzyme SOD, the three different forms of which are cytosolic copper-zinc SOD, extracellular copper-zinc SOD, and mitochondrial manganese SOD. The hydrogen peroxide generated is detoxified by catalase and glutathione peroxidase. If this step fails or is overloaded, and if iron is available, the Fenton reaction and the production of the deadly hydroxyl radical occur. Studies of animal models and more recent studies of asphyxiated human infants indicate that after hypoxia-ischemia, iron is released and is therefore relatively abundant. Studies of hypoxia-ischemia in the neonatal mouse indicate that detoxification of hydrogen peroxide is deficient in the immature brain. Thus mice made transgenic for copper-zinc SOD and therefore overexpressing this enzyme, when subjected to hypoxia-ischemia, exhibit decreased brain injury in the adult but increased brain injury in the perinatal period. This exacerbation of brain injury in the immature brain was associated with an accumulation of hydrogen peroxide, because catalase and glutathione peroxidase did not increase in activity in response to the insult (catalase increases after similar hypoxia-ischemia in the adult). Indeed, the levels of glutathione peroxidase decreased after hypoxia-ischemia, and importantly, in the normal animal levels already developmentally low in the perinatal period. Consistent with this, in normally developing rats (P7) rendered hypoxic-ischemic, an accumulation of hydrogen peroxide in the cortex was much greater than occurred with hypoxia-ischemia at later ages (P42). The particular importance of glutathione peroxidase was shown in two models derived from transgenic mice overexpressing glutathione peroxidase. Thus, in cultured neurons exposed to oxidative stress and in a neonatal hypoxic-ischemic model, glutathione peroxidase overexpression led to neuronal protection. Additional importance for glutathione is suggested by the finding in the hypoxic-ischemic 7-day rat that the crucial mitochondrial fraction of glutathione, necessary for glutathione peroxidase function, was markedly decreased after hypoxia-ischemia, with a nadir after 24 hours. Thus the data suggest that the normal immature brain has a limited capacity to detoxify hydrogen peroxide and with hypoxia-ischemia accumulates hydrogen peroxide, both because of this developmental lack and because of failure to respond with an adaptive increase in the antioxidant defense enzymes. With the hypoxia-ischemia–induced increase in iron, generation of the hydroxyl radical and brain injury is the result (see Fig. 13.37 ).

The propensity for oxidative neuronal injury in the immature brain relates not only to the deficient antioxidant defenses just described but also to several pro-oxidant characteristics . Thus, at baseline, the developing brain has a relatively high concentration of polyunsaturated fatty acids, especially in neuronal membranes, an excellent target for ROS, and a relatively high concentration of nonprotein-bound iron. Therefore, with exposure to conditions that lead to an increase in ROS (e.g., hypoxia-ischemia-reperfusion) and available iron, the balance strongly favors oxidative injury. Similar considerations apply to the increased oxidative injury observed after hypoxia-ischemia in developing animals or in asphyxiated infants when resuscitation is carried out with 100% oxygen rather than with room air (see Chapter 20 ).
Nitric Oxide and Reactive Nitrogen Species.
Particular importance for the synthesis of NO by NOS both in normal brain and under conditions of hypoxia-ischemia is now well established. At least three forms of NOS are recognized: a constitutive neuronal form (nNOS), a constitutive endothelial form (eNOS), and an inducible form (iNOS) found in astrocytes and microglia. The constitutive forms are activated by Ca 2+ , whereas iNOS stimulation appears to be Ca 2+ independent and is activated especially well by inflammatory stimuli (e.g., cytokines, lipopolysaccharide [LPS], and oxidative stress). Because NO is a diffusible gas, both its normal functions (i.e., cell signaling and neurotransmission) and its deleterious actions (i.e., neurotoxicity) appear to be mediated by synthesis and then diffusion to intracellular sites and to adjacent cells.
Under normal conditions, NO has multiple cellular effects. Many of these, but not all, are initiated by guanylate cyclase activation with the subsequent production of cyclic guanosine monophosphate and protein phosphorylation. This activation occurs when NO binds to the heme group of guanylate cyclase. Other biological effects of NO relate to action of its metabolites. Thus formation of a nitro group (NO 2 ) allows the nitration of proteins, especially at tyrosine residues, to form nitrotyrosine. Formation of nitrosonium ion (NO + ) allows nitrosylation of thiol residues on proteins, especially the cysteine sulfhydryl group. Formation of ONOO − and other reactive intermediates, including the hydroxyl radical, leads to oxidation of multiple amino acid residues, including the formation of nitrotyrosine. The biological effects of these actions of NO include modulation of cell proliferation, apoptosis, mitochondrial energy metabolism, and signal transduction.
Under pathological conditions of hypoxia-ischemia-reperfusion , an increase in cytosolic Ca 2+ is an early event (see earlier). A particularly important effector for the increase in Ca 2+ is activation of the NMDA receptor (see later). The effects on NO metabolism are multiple and sequential ( Fig. 13.38 ). Thus, in the first few minutes, the activity of Ca 2+ -dependent eNOS in endothelial cells is increased to produce vasodilation and to replenish substrate supply. The activity of Ca 2+ -dependent nNOS in neurons also is increased, and initially this increase may help improve blood flow because of the presence of nNOS in perivascular nerves. However, the principal effect of induction of nNOS in neurons is the generation of NO that diffuses to adjacent neurons. Under conditions of oxidative stress with abundant superoxide anion ( ), NO reacts quickly with
to form the particularly toxic RNS, ONOO − . Indeed, the affinity of
for NO to form ONOO − greatly exceeds the reaction of
with SOD, and thus ONOO − formation is greatly favored. This compound leads to neuronal death by multiple mechanisms, including especially mitochondrial impairment , energy depletion, and further failure of Ca 2+ -homeostasis (see earlier). Glutamate activation of the NMDA receptor is a major cause of the initial increase of cytosolic Ca 2+ that activates nNOS and leads to this deleterious cascade. Those NMDA receptor–containing neurons that express nNOS are resistant to the deleterious effects of hypoxia-ischemia and excitotoxicity (see later).

A later result of hypoxia-ischemia-reperfusion is the activation of iNOS , principally in astrocytes and microglia, with a resulting large, sustained increase in NO production, over many hours to days, depending on the experimental model studied (see Fig. 13.38 ). This activation occurs in parallel with inflammatory responses and is based on the activation of specific cell surface receptors for cytokines (tumor necrosis factor-alpha [TNF-alpha], interferon-gamma, and interleukin-1beta [IL-1beta]) and on the intracellular effects of ROS. The deleterious effects of this increase in NO are mediated primarily through formation of ONOO − , as discussed earlier. The possibility of a neuroprotective role of NO, mediated by NO + , has been raised, because S -nitrosylation at critical thiols on the NMDA receptor’s redox modulatory site leads to downregulation of channel activity. The biological impact of such a neuroprotective role currently is unclear.
In perinatal models of asphyxia or hypoxia-ischemia , evidence for both the neurotoxic effects and the beneficial vascular effects of NO synthesis have been obtained. a
a References .
Evidence for neurotoxic effects of NOS activation has consisted particularly of demonstration of neuroprotection by specific inhibitors of the synthase (e.g., nitrosoarginine derivatives). Although the data are not completely consistent, on balance the scheme shown in Fig. 13.38 depicts the major effects mediated by stimulation of the several forms of NOS. Particular importance of NO and RNS during development in part is suggested by the observation that the relative resistance of NOS-expressing neurons to NMDA-mediated toxicity is lost after the neonatal period in the developing rat.Role of Excitatory Amino Acids
A remarkable series of studies over the past several decades has revolutionized the understanding of the role of excitatory amino acids, particularly glutamate, as the mediators of neuronal death under conditions of hypoxia-ischemia. Before discussion of these studies, the normal aspects of glutamate biology at the excitatory synapse are reviewed.
Normal Features.
The relationships at the glutamate synapse among the presynaptic nerve ending, the postsynaptic dendrite, and the associated astrocyte are shown in Fig. 13.39 . Only the ionotropic receptors are shown in Fig. 13.39 (as discussed in the next paragraph). Glutamate release is provoked by the influx of Ca 2+ into the presynaptic nerve ending. Depolarization of the postsynaptic dendrite is related to Na + entry. The action of glutamate is terminated by potent, high-affinity, Na + -dependent reuptake mechanisms in both astrocytes and presynaptic nerve endings. Although the transporters per se are not energy dependent, energy failure, as with hypoxia-ischemia, leads to disruption of Na + -K + ionic gradients across the plasma membrane because of failure of the ATP-dependent Na + -K + pump; the results are impairment of the Na + -dependent glutamate transporters and ultimately reversal of transporter function. In the astrocyte, the ATP-dependent enzyme, glutamine synthetase, uses ammonia and glutamate to form glutamine, which diffuses to the presynaptic nerve ending to regenerate glutamate on removal of this second amino group (see Fig. 13.39 ). ATP depletion also causes this mechanism to fail, and thus clearly ATP depletion results in failure of the major reuptake and removal mechanisms and leads to accumulation of extracellular glutamate and to excitotoxicity (see the following discussion).

These mechanisms of reuptake and removal must be highly efficient, because although the intracellular concentration of glutamate is extraordinarily high (i.e., 5 to 10 mmol/kg), the extracellular concentration is approximately 1000-fold less (i.e., in the low micromolar range or perhaps lower). The high concentration of glutamate in neurons implies a large release of glutamate into the extracellular space when cell death occurs, an occurrence that is relevant not only to amplification of primary excitotoxic cell death, as occurs with hypoxia-ischemia, but also to the development of secondary excitotoxic cell death from other types of injuries to neurons (e.g., trauma). In addition, release of glutamate from astrocytes may be even more marked than from neurons, under ischemic conditions.
Glutamate acts at both ionotropic and metabotropic receptors ( Table 13.24 ). Three of these are ionotropic (i.e., are linked to ion channels). The NMDA receptor is linked to an ion channel for Ca 2+ , the AMPA receptor is linked to a channel for Na + entry, and the kainate receptor is linked to a channel for Na + entry. The AMPA receptor is rendered Ca 2+ impermeable by the presence of one of its four subunits—namely, the GluR2 subunit. This subunit is relatively sparse during early development both in rodent and human neurons, and this feature renders the AMPA receptor in immature neurons Ca 2+ permeable. This feature may underlie the involvement of AMPA receptors in the hypoxic-ischemic or glutamate-induced death of immature neurons (see later discussion). Although the NMDA receptor often is considered the most crucial for the excitotoxic effects of glutamate in developing neurons, data suggest comparable importance for the AMPA receptor (see later). Agents that increase or decrease glutamate activation at the synapse mediated by the NMDA receptor-channel complex are shown in Table 13.25 . A fourth glutamate receptor type is metabotropic (i.e., is coupled through a guanosine triphosphate binding protein [G protein] to an enzyme producing a second messenger, phospholipase C, for phosphoinositide hydrolysis). The resulting products, diacylglycerol and inositol triphosphate, function as second messengers, the former activating protein kinase C, which has many cellular effects, and the latter promoting Ca 2+ mobilization from the endoplasmic reticulum (see Fig. 13.35 ).
CONDITION | CEREBRAL BLOOD FLOW a,b (PERCENTAGE OF CHANGE) |
---|---|
Hyperventilation | |
30 min | −36 |
6 h | −12 c |
After hyperventilation | |
30 min | +210 |
6 h | +226 |
a No effect on cerebral metabolic rate for oxygen was observed at any time.
b Blood flow to cerebral hemispheres and midbrain.
c Not significantly different from zero; all other numbers different from control at P < .05 level.
Initially |
Redistribution of cardiac output so larger proportion enters brain |
Increase in cerebral blood flow |
Loss of cerebral vascular autoregulation |
Later |
Diminution of cardiac output; hypotension |
Decrease in cerebral blood flow |
The normal ontogeny of glutamate receptors is relevant to normal brain development and to the vulnerability of immature brain regions to excitotoxic cell death with hypoxia-ischemia. Detailed earlier studies of the development of binding sites for NMDA and non-NMDA receptor agonists in the rat showed a striking increase in the early phases of brain development. Later work, largely based on immunocytochemical and in situ hybridization studies, demonstrated the marked developmental increase more clearly, with peak values for the NMDA and AMPA receptors that exceed those in the adult brain. The peak values for NMDA and AMPA receptors were reached at only slightly different ages, with the NMDA peak preceding the AMPA peak. The timing of these peaks in the rat correlated with the perinatal period for the human brain . This can be depicted in Fig. 13.40 and is discussed with relevance to preterm brain injury ( Chapter 14 , Chapter 15 , Chapter 16 ) and term brain injury ( Chapter 18 , Chapter 19 , Chapter 20 ).

The molecular characteristics of the NMDA and AMPA receptors also are developmentally regulated, and the major characteristics of the developing receptors indicate enhanced Ca 2+ influx. Thus, regarding the NMDA receptor, the relative expression of the NR2B subunit compared with that of the NR2A subunit is increased in the immature brain versus that of the adult. NMDA receptors containing predominantly NR2B exhibit increased duration of NMDA receptor–mediated excitation and increased Ca 2+ influx . With regard to the AMPA receptor, in the immature brain the expression of the GluR2 subunit , which renders the AMPA receptor Ca 2+ impermeable, as in the mature brain, is relatively low . Thus the large numbers of AMPA receptors in developing neurons are largely permeable to Ca 2+ .
The transient, dense expressions of these ionotropic glutamate receptors of enhanced functional capabilities have implications not only for their role in normal development but also in determining neuronal death with hypoxia-ischemia (see later). The role of glutamate receptor activation and Ca 2+ influx relates to such processes as regulation of neurite outgrowth, synapse formation, cell death, selective elimination of neuronal processes, and functional organization of neuronal systems. However, in addition, these transient dense expressions of glutamate receptors of enhanced functional capabilities may become the unintended mediators of neuronal death with hypoxia-ischemia (see later discussion). Moreover, the likelihood that these principles apply to the developing human brain is supported by the demonstration of early overexpression of glutamate receptors in human hippocampus, cerebral cortex, deep nuclear structures (i.e., basal ganglia and thalamus), and certain brain stem nuclei—regions vulnerable to hypoxic-ischemic injury in the newborn (see Chapter 14 , Chapter 15 , Chapter 16 , Chapter 17 , Chapter 18 , Chapter 19 , Chapter 20 ).
Role of Glutamate in Hypoxic-Ischemic Cell Death in Cultured Neurons.
The critical role for glutamate in the mediation of hypoxic-ischemic neuronal death is established by a large body of experimental information, as summarized in Table 13.26 . a
a References .
The essential neurotoxicity of glutamate was shown initially in cultured neurons and subsequently in other in vitro and in vivo models (see later).Increase in cerebral blood flow beginning within minutes after the insult and lasting for up to several hours |
Decline in cerebral blood flow toward baseline or lower, with hypotension, following initial hyperemia |
“Delayed” increase in cerebral blood flow (“delayed” hyperemia) beginning between 12 and 24 h and lasting many hours and attenuated by nitric oxide synthase inhibitors |
Delayed cerebral hyperemia correlating with impaired mitochondrial oxygenation, “secondary” energy failure, and neuropathological injury |
The crucial initial observation was that cultured hippocampal neurons, obtained from the fetal rat, were resistant to prolonged anoxia before synapse formation occurred in the cultures, but they were very sensitive to the same anoxic insult after synaptogenesis was well developed. Thus, such mature cultures markedly deteriorated in the absence of oxygen. However, when synaptic activity in these mature cultures was blocked by the addition of high concentrations of magnesium, no effect of anoxia on the cultured neurons occurred ( Fig. 13.41 ). Thus the data demonstrated that synaptic activity resulted in neuronal death with oxygen deprivation. This protection by synaptic blockage with magnesium was shown later in a hippocampal slice preparation in which neuronal death could be produced in the CA1 region under anoxic conditions.

Because glutamate (as in hippocampus in vivo) was presumed to be the neurotransmitter mediating the synaptic activity in the experiments with the cultured hippocampal neurons and the slice preparation, a nonspecific postsynaptic blocker of glutamate was investigated to prevent the hypoxic neuronal death in culture. This agent protected neurons dramatically from anoxia ( Fig. 13.42 ). The particular role of glutamate synapses in hippocampal neuronal death was supported further shortly thereafter by the demonstration that hypoxic-ischemic neuronal injury could be prevented in vivo by prior section of glutamatergic afferents to the CA1 region.

The mechanisms of glutamate-induced neuronal death in cultured neurons were elucidated next. a
a References .
Two basic mechanisms were identified. One of these is rapid cell death that occurs in minutes and is initiated by glutamate receptor activation, Na + entry through all three ionotropic receptors, passive influx of Cl − down its electrochemical gradient with water following, and ultimately cell swelling and lysis. A second variety, so-called delayed cell death, occurring over many hours, is initiated principally by activation of the NMDA and immature (GluR2-deficient) AMPA receptors, with influx of Ca 2+ (as well as Na + ) and a series of Ca 2+ -mediated events to cell death (see Table 13.22 ). Delayed cell death appears to be the crucial form of neuronal death in vivo, and the importance of the NMDA and GluR2-deficient AMPA receptors and Ca 2+ influx is well established by studies of specific blockers of these receptors in cultured cells, brain slices, and in vivo models (see later discussion). bb References .
The data support the scheme shown in Fig. 13.43 . Note the particular involvement of the NMDA and immature GluR2-deficient AMPA receptors. Not shown in the figure is the accentuation of the increase in cytosolic Ca 2+ by Na + influx through all three ionotropic receptors, membrane depolarization, and opening of voltage-dependent Ca 2+ channels. Sustained membrane depolarization also leads to failure of glutamate uptake mechanisms and to sustained glutamate release . Thus cyclical internal amplification with multiple vicious cycles likely becomes operative.
Relevance of Glutamate-Induced Excitotoxicity to Hypoxic-Ischemic Injury in Vivo.
The relevance of the glutamate excitotoxic mechanisms to the in vivo situation is now clear. a
a References .
The first body of evidence establishing this relevance showed that extracellular glutamate concentrations in vivo increase manifold with hypoxic-ischemic insults. Such increases have been documented in perinatal animal models as well as in adults, although glutamate increases tend to be somewhat less in the former models than in the latter. bb References .
However, studies using microdialysis have documented the accumulation of extracellular glutamate in the brain of asphyxiated fetal sheep and of hypoxic-ischemic immature rats to concentrations of approximately 500 µmmol/L, concentrations easily sufficient to cause neuronal death in cultured cells. Moreover, glutamate concentrations in the cerebrospinal fluid of asphyxiated human newborns are markedly greater than concentrations in normal newborns . The reasons for the increase of extracellular glutamate with hypoxic-ischemic insults relate to impaired uptake of glutamate and to excessive release. The impaired uptake is related to defective operation of the high-affinity, Na + -dependent glutamate transporters in neurons and astrocytes (because of the failure of the ATP-dependent Na + -K + pump and loss of the Na + -K + ionic gradient across the plasma membrane) and to the defective function of the ATP-using glutamine synthetase reaction in astrocytes. The transporter function also is disrupted by ROS and RNS and by cytokines (e.g., TNF-alpha), released by activated microglia. The excessive release of glutamate relates to at least five factors. The first of these is the persistent membrane depolarization resulting from the failure of the ATP-dependent Na + -K + pump. (The destruction of gamma-aminobutyric acid neurons by hypoxia also may contribute to the excessive excitation and release of glutamate.) The second factor of critical importance is the reversal of glutamate transport because of the loss of the Na + -K + ionic gradient and elevated intracellular Na + levels. Indeed, this factor may be most important for the sustained release of glutamate into the extracellular space. A third factor is the rapid blockade of inhibitory synaptic transmission with relative preservation of excitatory synaptic transition with anoxia in the immature versus adult animal. A fourth factor promoting the excessive release of glutamate is the acute development of epileptic phenomena after hypoxia in the immature (but not mature) animal. A fifth factor is glutamate release from microglia, a process enhanced in an autocrine manner by TNF-alpha released by microglia with diverse inflammatory stimuli.The second body of evidence delineating the relevance of glutamate to the in vivo situation is the demonstration in a wide variety of perinatal models of hypoxia-ischemia that glutamate is toxic to neurons in vivo and that this toxicity is particularly marked in the immature versus the mature animal. c
c References .
In general, in the immature animal, the most toxic glutamate analogue is NMDA; AMPA is slightly less toxic, and kainate is the least toxic. dd References .
The approximate time of peak sensitivity in the rat is 6 days for NMDA and 9 to 10 days for AMPA. The particular vulnerability of the brain of the immature animal to hypoxia-ischemia and the importance of the NMDA receptor in this ontogeny of vulnerability are illustrated by the similar developmental profiles of hypoxic-ischemic neuronal death and NMDA-mediated neuronal death ( Fig. 13.44 ). A similar relationship has been shown for the GluR2-deficient AMPA receptor for the rat. Presumably, these particular vulnerabilities of the immature animal relate at least in part to the transient dense expression of NMDA and GluR2-deficient AMPA receptors during brain development (see earlier discussion).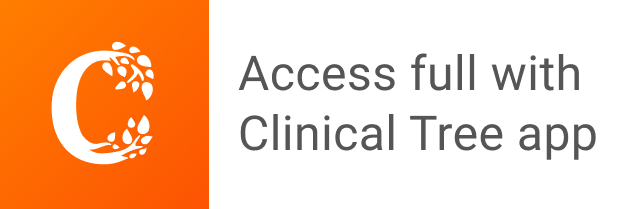