Pathophysiology of Epileptiform Activity
L. John Greenfield Jr
Sang-Hun Lee
Much of our attention as EEGers is devoted to the identification and localization of spikes and seizures. Atlases, primers and texts of EEG interpretation provide a wealth of information to guide seizure identification, but often the diagnosis is based on the same principle as Justice Potter Stewart’s maxim for identifying obscenity in Jacobellis v. Ohio: “I know it when I see it.”1 Virtually all of the mathematical seizure detection algorithms currently in use are based on empiric observations of EEG activity that occurs contemporaneously with behavioral seizures or resembles the electrical activity we see during such behaviors. Ideally, we should be able to derive the parameters for identifying electrographic seizures from a detailed understanding of the underlying neuronal pathophysiology that generates abnormal rhythmic activity, disrupting normal brain circuit functions and behaviors. Unfortunately, we are not there yet. In many cases, however, we have at least a rudimentary knowledge of the neurons and brain structures involved in seizure generation. This chapter will review what we know about how seizures are generated and how that translates into the interictal and ictal patterns we observe in EEG recordings. You may want to review the information in Chapter 1 on Basic Neuroscience of EEG before tackling this topic.
ANATOMICAL SUBSTRATES OF FOCAL EPILEPTIFORM ACTIVITY AND SEIZURES
The cytoarchitecture and connectivity of the cerebral cortex and hippocampus function to generate rhythmic brain activity. Defects in the rhythm-generating circuits are responsible for epilepsy and possibly other neurologic and psychiatric disorders.
The hippocampus has served as a robust model system for studying epileptiform activity due to its simplified architecture and its proclivity to generate seizures. The basic structure of the hippocampus, when laid lengthwise and sliced like a loaf of bread, reveals interlocking horseshoes consisting of the cell body and dendritic “molecular” layers of the dentate gyrus (DG) and the cornu ammonis (CA) layers of the hippocampus proper. A trisynaptic excitatory pathway passes through the hippocampus within the plane of the slice, allowing unidirectional information throughput (see Fig. 10.1).2 Excitatory input from the entorhinal cortex (EC) passes into the DG via the perforant pathway and synapses onto DG granule cell neurons. The axons of DG granule cells, called mossy fibers, project through the dentate hilus (the space enclosed by the horseshoe of granule cells) to the pyramidal cells of the CA3 region. The axons of the CA3 cells, called Schaffer collateral fibers, in turn pass around the hippocampal horseshoe to the pyramidal cells of the CA1 region. The axons of CA1 pyramidal cells then pass information either directly back to the EC or via the subiculum, a transitional cortical layer. Recurrent excitatory connections within the CA3 cell layer reinforce the excitatory throughput, and lead to discrete bursting of APs, a behavior that can produce lasting synaptic strengthening at downstream synapses by the mechanism known as LTP.
The positive feedback loop (from EC to DG to CA3 to CA1 [to subiculum] to EC) would produce runaway excitation in the absence of inhibitory feedback. To prevent this, feedback and feed-forward inhibitory loops exist at multiple points in the circuit. In the DG, the mossy fibers from dentate granule neurons excite “mossy cells” in the dentate hilus, which in turn excite inhibitory basket cells that strongly inhibit dentate granule cells in a “surround” fashion. This inhibitory system has been called the “dentate gate”3,4,5 as it limits and focuses excitatory input into the hippocampus. Elsewhere in the hippocampus, and throughout the cerebral cortex, GABAergic interneurons with varied morphologic, neurochemical, and electrophysiological properties are critical for maintaining the balance of excitation and inhibition required to generate normal brain rhythms, and their dysfunction is implicated in disorders including epilepsy, autism, and schizophrenia.6
REVIEW
10.1: List the major components of the hippocampal trisynaptic circuit.
View Answer
10.1: Entorhinal cortex (EC) projects to the dentate gyrus (DG) via the perforant pathway. DG axons innervate CA3 pyramidal neurons in the hippocampus proper, as well as mossy cells in the dentate hilum that project back to inhibit DG granule neurons via basket cells, forming the “dentate gate.” CA3 projects to CA1 via Schaffer collaterals. CA1 projects out of the hippocampus to subiculum and EC.
10.2: What is the major neurotransmitter responsible for hippocampal synaptic inhibition?
Pathophysiology of Temporal Lobe Epilepsy
In both human temporal lobe epilepsy (TLE) and animal models, a number of structural and physiological changes in the hippocampus likely contribute to the generation of seizures. In human TLE, there is extensive damage to the region of the dentate hilus and CA3 and CA1 regions with both gliosis and neuron loss, known as mesial temporal sclerosis.7,8,9 The loss of dentate hilus mossy cells and CA3 pyramidal neurons and their inputs to dentate basket interneurons may contribute to reduced inhibition at the dentate gate, which is known as the dormant basket cell hypothesis.10,11 However, more recent studies have suggested that the regulation and role of hippocampal interneurons and mossy cells in TLE are far more complex. For example, elegant studies by Bui et al.12 recently showed that optogenetic activation of surviving mossy cells prevented spontaneous seizure progression from an electrographic stage into overt behavioral seizures (Racine stage 4-5, the rodent equivalent of generalized tonic-clonic seizures) in a well-established mouse model of TLE. Optogenetic inhibition of surviving mossy cells increased behavioral seizures, suggesting that surviving mossy cells plays a protective role in TLE.12 Another major structural change is the recurrent sprouting of dentate granule cell mossy fiber axons to supply recurrent excitation to dentate granule cell proximal dendritic synapses vacated by the loss of mossy cells and commissural axon inputs.13,14,15 In addition, adult newly generated dentate granule neurons with aberrant dendritic morphology may drive excessive neuronal activity and trigger seizures.16,17 At the subcellular level, changes in the number and subunit composition of inhibitory GABAA receptors on CA1 pyramidal neurons may reduce inhibitory drive and promote seizures.18,19 It remains unclear which of these mechanisms are critical for epileptogenesis and which may be adaptive changes in response to epileptic activity. Seizures can occur despite inhibition of DG mossy fiber axonal sprouting,20 suggesting that other mechanisms are at least as important or that under some conditions the dentate gate may be bypassed.5 However, Krook-Magnuson et al. demonstrated that optogenetic restoration of the dentate gate through selective inhibition of granule cells was sufficient to inhibit spontaneous seizures in a mouse model of TLE, confirming the underlying concept that the DG is critical for seizure suppression.5
The epilepsy-associated structural changes noted above contribute to pathologic electrophysiological changes at the single cell, local field, and EEG levels. The normal hippocampus typically generates θ frequency activity in concert with the overlying EC and parahippocampal gyrus. In the rat, hippocampal θ activity occurs during exploratory behaviors such as sniffing, rearing, and walking and during rapid eye movement (REM) sleep. Intracellular recordings performed during simultaneous field potential measurements demonstrate that interneuron firing is phase linked to θ oscillations.21,22 By contrast, hippocampal pyramidal cells are typically silent or fire at specific spatial locations associated with a neuronal map of the spatial environment, which is critical for episodic memory processing.23,24,25 In the absence of θ activity, intermittent sharp waves of 40- to 120-ms duration are observed in the CA1 stratum radiatum, typically during feeding, behavioral immobility, and slow-wave sleep.26,27 Hippocampal sharp waves occur with a frequency of 1 per minute to about 3 per second and reflect the synchronous discharge of a large number of CA3 pyramidal neurons and consequent depolarization of CA1 pyramidal cells. Hippocampal sharp waves frequently have superimposed gamma (γ) frequency “ripple” oscillations (known as spike-wave-ripple complexes) and intracellular recordings in CA1 hippocampal pyramidal cells display bursts of 200-Hz oscillations.28 The sharp wave appears to be generated in the CA3 region by a highly synchronized discharge of CA3 pyramidal neurons, which then projects via Schaffer collaterals into the CA1 region
and activates rhythmic 200-Hz (“ripple”) inhibitory postsynaptic potentials (IPSPs) in CA1 pyramidal neurons via feed-forward inhibition.29 These high-frequency IPSPs are likely mediated by fast-spiking, parvalbumin-positive basket cells (PVBCs).30 Functionally, the sharp-wave-ripple discharges appear to be critical for spatial learning and memory possibly by replaying sequenced neuronal activity in a compressed manner.31 In the setting of decreased inhibition or excessive synchronization, they may also be related to the physiological mechanisms that are augmented to generate interictal EEG spikes in the temporal lobe, though the physiological sharp wave discharges are clearly distinct from epileptiform activity. They may also contribute to high-frequency oscillations (HFOs), which we will discuss in greater detail below.
and activates rhythmic 200-Hz (“ripple”) inhibitory postsynaptic potentials (IPSPs) in CA1 pyramidal neurons via feed-forward inhibition.29 These high-frequency IPSPs are likely mediated by fast-spiking, parvalbumin-positive basket cells (PVBCs).30 Functionally, the sharp-wave-ripple discharges appear to be critical for spatial learning and memory possibly by replaying sequenced neuronal activity in a compressed manner.31 In the setting of decreased inhibition or excessive synchronization, they may also be related to the physiological mechanisms that are augmented to generate interictal EEG spikes in the temporal lobe, though the physiological sharp wave discharges are clearly distinct from epileptiform activity. They may also contribute to high-frequency oscillations (HFOs), which we will discuss in greater detail below.
REVIEW
10.3: What structural physiological changes in the hippocampus have been associated with seizures in animal models of temporal lobe epilepsy?
View Answer
10.3: Structural changes in the hippocampus include damage and cell loss in areas CA1, CA3, and dentate hilum, recurrent sprouting of DG granule cell axons onto dendritic regions vacated by lost mossy cells, aberrant dendritic morphology of newborn DG neurons, and altered GABAA receptor subunit composition.
10.4: Describe the EEG patterns generated by the hippocampus and the states/behaviors associated with each pattern?
View Answer
10.4: Theta activity is recorded during exploratory behaviors (sniffing, rearing, walking) and during REM sleep (which may recapitulate waking behaviors as a form of memory consolidation). Sharp wave-ripple discharges are recorded in the stratum radiatum during feeding, behavioral immobility, and slow-wave sleep.
Pathophysiology of Focal Interictal Spike-and-Wave Discharges
The EEG hallmark of focal epilepsies is the interictal spike. Spikes are typically brief (70-200 ms), focal, often high-amplitude waveforms that are most often arrhythmic, occurring independently of other brain rhythms and tending to locally disrupt them. They can occur on a normal background or in the context of focal delta (δ) slowing in the same region. The intracellular correlate of the interictal spike is a high-amplitude depolarizing potential associated with a burst of action potentials (APs), known as the paroxysmal depolarizing shift (PDS).32,33 An example of an intracellularly recorded PDS in a CA3 hippocampal neuron is shown in Figure 10.2. Early animal studies found that penicillin, bicuculline, or other agents that blocked GABAA receptors, when applied to the cortical surface in vivo or the hippocampal slice in vitro,34 resulted in spike-and-wave discharges on EEG that corresponded to PDS events recorded intracellularly. The PDS is essentially a high-amplitude excitatory postsynaptic potential (EPSP), generated by synaptically driven34 synchronous depolarization and bursting of CA3 pyramidal neurons and mediated by recurrent activation of non-NMDA (AMPA, kainate) and NMDA glutamatergic ionotropic receptors. The bursting activity resulting from the high-amplitude synaptic depolarization is due to influx of extracellular Ca2+ through NMDA receptors and voltage-gated calcium channels, with subsequent opening of voltage-gated Na+ channels and generation of repetitive or bursting Na+ channel-dependent APs. The burst of APs results in rapid spread of depolarization and activation of other excitatory cells through the hippocampus and parahippocampal gyrus, generating the local field potential that underlies the spike component of the spike-and-wave discharge seen on EEG.35
After the high-amplitude depolarization of the PDS, the subsequent prolonged hyperpolarizing afterpotential is mediated by fast GABAA receptor/chloride channels, slower metabotropic GABAB receptors, and Ca2+-dependent K+ channels activated by the influx of Ca2+ during the PDS. The activation of Ca2+-dependent K+
channels can prolong the hyperpolarization to 1 second or more.36 The large spatial extent of the hyperpolarizing afterpotential is driven by PDS-induced activation of GABAergic interneurons, predominantly basket cells that produce strong perisomatic inhibition on a large number of neighboring principal neurons, creating an “inhibitory surround” that broadly silences excitatory activity for up to hundreds of milliseconds. At the macroscopic EEG level, the afterhyperpolarization is the slow “wave” of the spike-and-wave complex, and the silencing of excitatory activity by this wave of inhibition explains why spike-and-wave complexes disrupt the local background EEG rhythm.
channels can prolong the hyperpolarization to 1 second or more.36 The large spatial extent of the hyperpolarizing afterpotential is driven by PDS-induced activation of GABAergic interneurons, predominantly basket cells that produce strong perisomatic inhibition on a large number of neighboring principal neurons, creating an “inhibitory surround” that broadly silences excitatory activity for up to hundreds of milliseconds. At the macroscopic EEG level, the afterhyperpolarization is the slow “wave” of the spike-and-wave complex, and the silencing of excitatory activity by this wave of inhibition explains why spike-and-wave complexes disrupt the local background EEG rhythm.
REVIEW
10.5: What is the physiological basis of the paroxysmal depolarizing shift (PDS)?
View Answer
10.5: The PDS is a large synchronous postsynaptic EPSP mediated by glutamate at NMDA and non-NMDA receptors, which activates bursts of Na+-dependent action potentials. This triggers recurrent/feedback GABAergic synaptic inhibition in a surround fashion, as well as prolonged opening of Ca2+-dependent K+ channels, terminating the depolarization and causing subsequent hyperpolarization.
10.6: What components of the PDS underlie the spike-and-wave on EEG?
View Answer
10.6: The depolarizing phase of the PDS corresponds to a spike discharge on EEG, and the subsequent surround inhibition results in the aftergoing slow wave on EEG.
In order to generate a signal large enough to be detected by surface EEG, a relatively large area of the cortex must be activated. Modeling the transmission of electrical signals across the skull and scalp, Cooper et al.37 proposed that “at least 6 sq. cm must be involved in synchronous or near synchronous activity before the scalp EEG is observed.” More direct results of combined subdural (cortical surface) and scalp EEG recordings by Tao et al.38 found that cortical spike sources of 10 cm2 or more commonly resulted in scalp-recordable interictal spikes. This corresponds to a circle with a radius of 1.8 cm or the simultaneous activation of more than 5000 cortical columns, each composed of thousands of neurons. Prominent scalp spikes on surface EEG were associated with the activation of 20-30 cm2 of the cortex, an area covering as much as 70% of the temporal lobe gyral cortex. Clearly, massive synchronization of cortical activity is involved.
REVIEW
10.7: How much cortical area must be activated to generate an observable spike on EEG?
View Answer
10.7: About 10 cm2 of the cortex must be synchronously activated to generate an EEG spike.
Relationship of Spikes and Rhythmic Slowing
Focal intermittent slowing in a region that generates spike discharges may represent interictal epileptiform activity with insufficient cortical synchrony, spatial extent, or scalp proximity (eg, due to arising in the mesial temporal lobe) to produce an observable spike at scalp electrodes, with the slow waves arising from the potent, more spatially extended, and much longer-lasting inhibitory surround. Rhythmic focal slowing, particularly involving the temporal lobe (temporal intermittent rhythmic delta activity, TIRDA), is so commonly associated with epileptiform activity, focal pathology, and seizures that it is felt to be a forme fruste of epileptiform activity with lateralizing if not localizing value.39,40,41 As noted above, such activity appears to be generated by rhythmic spike-and-wave discharges in which the spike component is not observable. The specificity for seizures is also reasonably strong for occipital intermittent rhythmic delta activity (OIRDA), which occurs almost exclusively in children with epilepsy,42,43 but not in the frontal intermittent rhythmic delta activity (FIRDA) where it is nonspecific and usually associated with encephalopathy.44,45
Rhythmic or Periodic Spike-and-Wave Discharges
Focal interictal spike-and-wave discharges are usually sporadic, but may also occur periodically or rhythmically, as seen with periodic lateralized discharges (PLDs). The generation of such rhythms likely arises from the structure and physiology of the irritable focus itself. Using the cortical surface penicillin model, Witte36 noted that cortical neurons in the focus region have a high negative resting potential and are often silent until they are driven to paroxysmal bursting by synaptic input from neurons in the “inhibitory surround” region. Indeed, the firing patterns of neurons in the surround show markedly increased activity during the 100 ms just before the spike discharge and are silent immediately afterward for up to 1 second before resuming stochastic firing patterns. It is easy to see how this relationship between a central paroxysmal focus and an inhibited surround could generate rhythmic spike patterns. Neurons in the surround recovering from strong inhibition synaptically activate hyperexcitable neurons in the central epileptic focus and trigger a PDS, resulting in a focal paroxysmal discharge and strong inhibition in the surround neurons. When the recurrent inhibition dissipates, recovery from hyperpolarization triggers a new PDS in the focus. The period of the discharge cycle results from the refractory period of the neurons in the focus, which can last for up to several seconds due to the accumulation of calcium during the PDS and associated calciumdependent K+ currents. The timing of such periodicity nicely correlates with the 0.5- to 2-second period usually associated with PLDs and suggests that this rhythm is intrinsic to the epileptic focus, though the mechanism producing it is obviously different from the artificial scenario produced by focal application of penicillin. PLD discharges are often associated with focal irritative or structural lesions such as acute stroke, encephalitis, or brain tumors, consistent with the concept that the PLD epileptic focus is the result of acute or chronic cortical injury.46 Whether these discharges represent seizures in themselves has been long debated47 and veers from the purely scientific into philosophic territory. PLDs are often associated with electrographic and clinical seizures48 and can occur as a pattern associated with status
epilepticus.49 However, the concept that “PLED” discharges are epileptiform has been questioned, and the currently accepted term endorsed by the American Clinical Neurophysiology Society (ACNS) is lateralized periodic discharges (LPDs), discarding the “epileptiform” designation.50 Despite the official change in terminology, the association of this EEG pattern with seizures is strong. The connection becomes murkier with generalized periodic discharges and periodic triphasic waves, which are far less associated with seizures51 even though they often have an initial sharp component. These patterns are more often associated with metabolic disturbances or diffuse brain injury,52 particularly resulting from hypoxia-ischemia,53 where they confer a poor prognosis for survival and cortical recovery.54
epilepticus.49 However, the concept that “PLED” discharges are epileptiform has been questioned, and the currently accepted term endorsed by the American Clinical Neurophysiology Society (ACNS) is lateralized periodic discharges (LPDs), discarding the “epileptiform” designation.50 Despite the official change in terminology, the association of this EEG pattern with seizures is strong. The connection becomes murkier with generalized periodic discharges and periodic triphasic waves, which are far less associated with seizures51 even though they often have an initial sharp component. These patterns are more often associated with metabolic disturbances or diffuse brain injury,52 particularly resulting from hypoxia-ischemia,53 where they confer a poor prognosis for survival and cortical recovery.54
REVIEW
10.8: Describe a possible mechanism underlying lateralized periodic discharges (LPDs).
View Answer
10.8: LPDs could correspond to repetitive activation of an irritable seizure focus by neurons in the inhibitory surround, which causes a PDS that corresponds to the epileptiform discharge. The PDS results in surround inhibition, which lasts for up to several seconds but then dissipates, allowing the surround to reactivate the focus when inhibition wears off.
Repetitive Focal Spikes and Seizures
Using the focal cortical penicillin model, Witte and colleagues observed distinct patterns of epileptiform discharges: irregular, occurring from 1.7 to 4.8 seconds apart, “composed,” with several discharges occurring at intervals of about 300 ms separated by longer irregular intervals, regular 1-Hz spike discharges, and ictal evolving seizure discharges. These patterns occurred in an ordered sequence indicating that the different rhythms are activated by a progressive enlargement of the focus and duration of focal activity and suggest that different interictal discharge patterns can occur within the same brain regions and may represent characteristic “resonance” frequencies for specific brain regions susceptible to seizure generation. Changes in the pattern of spike discharges occur over the course of minutes to hours in the penicillin model and are specific to this model. However, such changes might be analogous to the gradual recruitment of surrounding tissues into epileptic circuits due to progressive loss of inhibition or synaptic learning mechanisms such as long-term potentiation. Such recruitment has been demonstrated in animals using the process known as “kindling,” with electrical pulse trains (usually in the hippocampus or amygdala) sufficient to produce a brief rhythmic afterdischarge (a train of spikes that continues after the end of the stimulus) but no behavioral change or sustained electrographic seizure. When repeated daily, each stimulation gradually produces longer and longer afterdischarges. Eventually, these produce increasingly severe behavioral seizures, beginning with freezing and staring, progressing through facial twitching automatisms and forepaw clonic jerking, to rearing and falling, the rodent equivalent of a generalized tonic-clonic seizure.55 The evolution of seizure semiology and severity seen in kindling has not been directly demonstrated in humans. However, many epilepsies gradually worsen in seizure frequency and severity over time, particularly when seizures are poorly controlled. Moreover, the latent period between brain injury and the onset of posttraumatic epilepsy may be 10 years or longer, suggesting that undetected subthreshold epileptiform activity may become progressively more severe until presenting years later with a clinical seizure. These observations provide indirect evidence that a similar phenomenon may occur in people with epilepsy.
Interictal to Ictal Transition
What is the relationship between spike-and-wave discharges and the sustained, evolving rhythmic activity that constitutes a seizure? The mechanisms underlying the transition from interictal to ictal state at the onset of a focal seizure are poorly understood. On scalp EEG, the onset of focal seizures is frequently associated with a reduction in the amplitude of background alpha or theta frequencies and a “flattening” of the EEG amplitude. As recorded with stereotaxic EEG depth electrodes or grid/strip subdural electrodes in patients with either TLE or extratemporal neocortical epilepsies, this loss of amplitude corresponds to the appearance of lowamplitude fast activity in the beta to low-gamma frequency range (15-40 Hz).56 This change in background may be preceded by either a reduction in interictal spike frequency or increased spike frequency, with discharges of higher than usual amplitude, often occurring in brief clusters. Low-voltage fast activity may be mediated by spatial desynchronization of cortical networks due to excessive inhibition, as seen after a high-amplitude spike discharge encompassing a broad area of the cortex.57
That initial hyperpolarizing stimulus may be produced by a strong and prolonged PDS. Early experiments by Ayala and colleagues32,35 using the topical penicillin model found that the seizure focus transitioned from sporadic interictal spike/PDS complexes to PDS discharges of increasing frequency and longer depolarizations, with reduction or loss of afterhyperpolarization, suggesting a change in the balance of excitatory and inhibitory synaptic drive. Their figure demonstrating the hypothesis that seizures are generated from a prolongation of the PDS (Fig. 10.3)32 set the stage for many subsequent investigations into ictal mechanisms. However, some neurons were strongly hyperpolarized even during clonic seizures, with reversal potential suggesting increased K+ conductance. Ayala et al. postulated that the hyperpolarization during the clonic seizure phase was related to the termination of the seizure, though it is also possible that hyperpolarization contributes to the spatial desynchronization of cortical rhythms that sets the stage for ictal onset.
The concept that seizures are the product of excess synchrony and a loss of the “balance of excitation and inhibition” is an oversimplification. The loss of normal cortical rhythms may allow pathologic rhythmic activity in the seizure focus to recruit neighboring and connected cortical regions into the pathologic evolving
rhythm that constitutes a seizure. Recruitment of additional brain areas into the seizure increases synchrony over the course of the seizure, which is maximal near the time of seizure termination.
rhythm that constitutes a seizure. Recruitment of additional brain areas into the seizure increases synchrony over the course of the seizure, which is maximal near the time of seizure termination.
REVIEW
10.9: What observations support the concept of “kindling” in human epilepsy?
View Answer
10.9: Some seizure disorders, like TLE, can worsen in severity over time, particularly when seizures are not well controlled, suggesting that seizures beget worse seizures. The long latent period between closedhead injury and onset of epilepsy is consistent with prolonged subclinical discharges that eventually evolve into seizures.
10.10: What EEG features are often seen at the onset of a focal seizure?
View Answer
10.10: At the onset of a focal seizure, there is often a “flattening” of the EEG amplitude with loss of theta and alpha activity, replaced by lowamplitude fast activity in the 15- to 40-Hz range, which may be preceded by a change (increase or decrease) in spike frequency. The loss of amplitude may represent spatial desynchronization of cortical networks, possibly due to excessive inhibition.
Seizure Termination
The mechanisms underlying seizure termination are also uncertain. As seizures progress, rhythms typically slow into the delta frequency range, consistent with greater spatial extent and longer reactivation pathways for spiraling cycles of recurrent excitation and inhibition. In the cat model of spontaneous spike-and-wave seizures, as in many focal-onset seizures in humans and other animal models, synchronous activity toward the end of the seizures slows to ˜1-Hz high-amplitude oscillations. Timofeev and Steriade found that these discharges were synchronized by EPSPs, but paced by intrinsic neuronal oscillations. Each cycle was initiated by hyperpolarization-activated depolarizing current (Ih) and then enhanced by voltage-gated Na+ and Ca2+ currents, which then activate hyperpolarizing potassium currents (IK). Eventually, Ca2+-dependent hyperpolarizing currents overwhelm the depolarizing effect of the Ih component of the oscillation, resulting in seizure termination.58,59 An alternative hypothesis is that pH changes induced by synchronous discharging of neurons during the periodic bursting typical of the late clonic seizure phase may promote synchronous postictal depression of principal cell activity via decoupling of gap junctions, leading to synchronization of inhibition, which contributes to seizure termination.63 In support of this mechanism, an extracellular alkaline shift has been observed in the piriform cortex of the isolated guinea pig brain associated with rhythmic interictal spikes, which correlated with interspike periodicity. It is likely that multiple electrophysiological and metabolic mechanisms contribute to both seizure onset and termination.
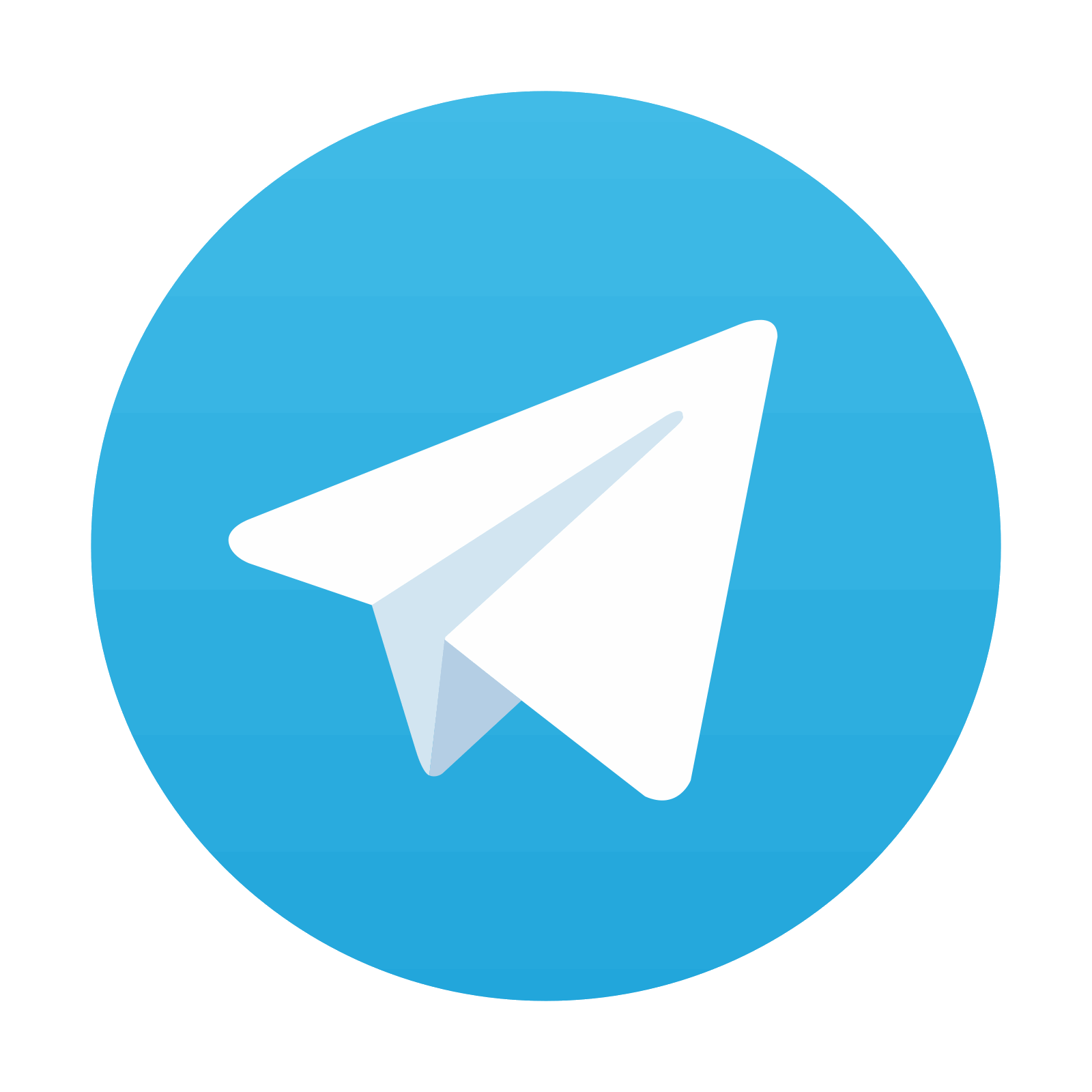
Stay updated, free articles. Join our Telegram channel
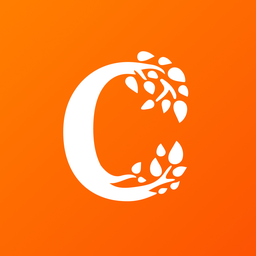
Full access? Get Clinical Tree
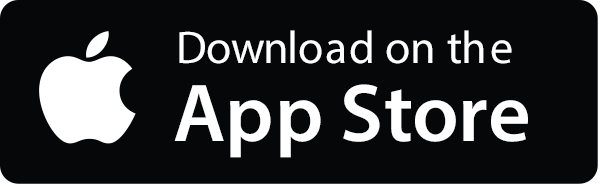
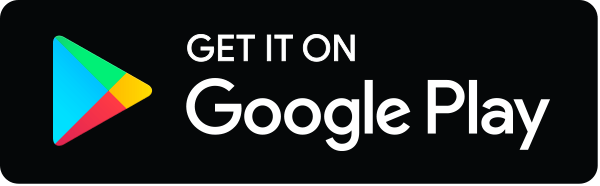