© Springer International Publishing Switzerland 2017
Norbert Weidner, Rüdiger Rupp and Keith E. Tansey (eds.)Neurological Aspects of Spinal Cord Injury10.1007/978-3-319-46293-6_1919. Pathophysiology of Traumatic Spinal Cord Injury
(1)
Paracelsus Medical University, Institute of Experimental Neuroregeneration, Spinal Cord Injury and Tissue Regeneration Center Salzburg (SCI-TReCS), Strubergasse 22, 5020 Salzburg, Austria
Abstract
Traumatic spinal cord injury (SCI) is a drama in two acts. The first part represents the trauma itself, causing the destruction of neural tissue, i.e., the elimination of neuronal and glial cells at the primary lesion site, as well as the transection of axons transiting through the lesioned area. Additionally, damage to the vascular system will provoke hemorrhage and the disruption of the blood–spinal cord barrier. Together, these damages will induce secondary cascades responsible for cell death, enlargement of lesioned area, and further loss of neurological functions. Edema will develop in the early ischemic period triggering a phase of glutamate excitotoxicity and ionic imbalance. The ensuing mitochondrial failure is thereafter responsible for an energy depletion and oxidative stress. The rapid inflammatory response to spinal cord injury is provided by the resident microglia, but foremost by the infiltrating neutrophils and macrophages. At the end of the acute phase, the lesioned area will get enclosed and stabilized by a fibroglial scar. This chapter reviews the sequence of pathophysiological processes occurring after traumatic spinal cord injury, which constitute targets for potential protective or regenerative interventions.
19.1 Introduction
Traumatic spinal cord injury (SCI) is an unexpected and devastating change in the life of affected individuals. Although the survival rates and the clinical management following spine injury significantly improved over the last century [1–3], no curative interventions are currently available despite intensive past and ongoing research efforts to restore lost tissues and neurological functions. To date, preservation of spared spinal cord tissue following trauma is the most important objective. Detailed knowledge on the pathophysiology and the sequence of events taking place following trauma is therefore crucial to develop adequate and optimized interventions in response to SCI. In this respect, an early surgical intervention for decompression of the spinal cord and mechanical stabilization of the spine represents the first treatment to circumvent additinal loss of spinal tissue (see chapter 7). Early decompression surgery might lead to a significant decrease in the length of acute hospital stay and the rate of complications [4].
Traumatic SCI can be seen as a drama in two acts. First, the mechanical trauma per se results in the destruction of neural parenchyma, which cannot be remediated. Mechanical injury directly disrupts axons, blood vessels (hemorrhage), and neural and glial cell membranes. The majority of primary injuries are associated with a compression due to dislocation or compression resulting in a blunt injury rather than an invasive transection (e.g., stab injury) [5, 6]. As a rule of thumb, the kinetic and the duration of the compression are the main determinants of the severity of the traumatic SCI [7, 8]. Thereafter, processes causing secondary damages are being successively activated, which lead to a propagation of the lesion within the neural tissue initially spared by the trauma. For technical reasons, the investigation of the pathophysiology of SCI has been much more extensively investigated in animal models (from rodents to nonhuman primates) than in human. Although this allowed for detailed temporal analysis and assessment of various pathological processes, the possibility of inherent differences with the human situation must be kept in mind.
This chapter reviews actual knowledge of the pathophysiology of SCI and describes the cascade of processes that might become targets for neuroprotective strategies and, eventually, regenerative therapies.
19.2 Primary Phase
In patients, traumatic SCI is provoked by physical forces acting on the spine, i.e., flexion, extension, rotation, distraction, compression, or a mixture of them. The resulting damages are referred to as the primary damages and correspond to a mechanical disruption of axons and other membranes of neural and glial cells and also in damage of blood vessels and micro-hemorrhages within the gray matter. In animal models, traumatic SCI is often simulated by direct contusion or compression of the spinal cord.
Recent epidemiological data revealed that most of the traumatic spinal cord injuries do not lead to a complete transection of the spinal cord (see chapter 1). Hence, even if the clinical manifestation following SCI suggests a “complete” functional loss, the segments above and below the level of injury remain connected by a few axons, which correspond to an anatomically incomplete or “discomplete” lesion [9, 10]. The extent of axonal sparing required for the maintenance of significant neurologic functions below the level of injury is not precisely defined. However, residual motor functions have been observed in patients with approximately 7–8 % of all former axons reaching below the injury level [1–3, 9]. Similarly, functional studies in animal models have suggested the requirement for an axonal sparing of 1.4–12 % across the lesion site [4, 11–13]. Although few percentages of residual spared fibers are sufficient for meaningful functionality, this low relative number represents a large amount of fibers in absolute number considering, for example, that 2–2.5 million afferent fibers are entering the dorsal roots at each side of the human spinal cord [5, 6, 14].
19.3 Secondary Phase
19.3.1 Immediate
Following the primary insult, the loss of neurons and glia and the disruption of axons cause a loss of function at and below the level of the injury. Within minutes, the spinal cord swells and occupies the whole spinal canal at the injury level. Increasing spinal cord pressure beyond the arterial blood pressure leads to secondary ischemia, one of the major triggers of secondary damages [7, 8, 15]. Moreover, further neurologic functions can be transiently affected due to the spinal shock [9, 10, 16, 17].
19.3.1.1 Vascular Damages and Ischemia
Freshly injured SCI patients are particularly vulnerable for systemic hypotension as a consequence of hypovolemia, neurogenic shock, and bradycardia [18]. This hypotension, conjugated with the loss of autoregulation of intraspinal blood flow and the increased interstitial pressure, results in a hypoperfusion of the spinal tissue at the lesion epicenter [19–21]. Paradoxically, some hyperperfusion was also detected in regions adjacent to the lesion site [21]. The deleterious impact of hypotension/hypoperfusion is substantiated by the correlation associating a higher mean arterial blood pressure in the acute phase of SCI with a higher degree of long-term neurological recovery [22].
The primary injury normally spares large-caliber vessels, such as the anterior spinal artery (see chapter 2 and 5) [23–25]. Nevertheless, intramedullary distal vessels are affected by occlusion and vasospasms following SCI leading to a central ischemia despite sparing of the major supplying arteries located at the surface of the spinal cord [25]. This hypoperfusion developing from the gray toward the white matter slows and eventually blocks the propagation of action potentials along axons, contributing therewith to the spinal shock [15]. The high metabolic rate of neurons within the gray matter makes them particularly sensitive to ischemia. In addition, damages of the small-caliber vessels compromise the blood–spinal cord barrier and lead to the extravasation of blood-borne molecules into the parenchyma, in cases of small lesions, or to the penetration of red blood cells in the cases of larger trauma with hemorrhage [26]. In this respect, the gray matter is more prone to be hemorrhagic than the white matter, because of its dense capillary network that is vulnerable to mechanical damage. Already 5 min after contusion injury, extravasation of labeled molecules is particularly pronounced in the well-vascularized gray matter [26].
Hemorrhages and swelling within the gray matter exacerbate mechanical damages and ischemia and promote spreading of necrotic cell death rostral and caudal from the initial site of injury [27]. Ischemia and necrosis trigger the inflammatory processes, such as the activation of microglia, recruitment of immune cells, and the secretion of pro-inflammatory cytokines, such as tumor necrosis factor (TNF)α and interleukin (IL)-β [28, 29]. Ischemia and necrosis also lead to an accumulation of extracellular glutamate that initiates the processes of excitotoxicity [30]. Paradoxically, following the ischemic period, the reperfusion of tissues surrounding the lesion is associated with an increased generation of detrimental free radicals.
19.3.1.2 Edema
Traumatic SCI induces the so-called cytotoxic, ionic, and vasogenic edemas. The intracellular compartment encloses approximately 70 % of the central nervous system (CNS) fluid and is richer in K+ and much poorer in Na+ and Ca2+, as compared to the extracellular/interstitial compartment [31]. Under physiological condition, the Na+/K+ ATPase and the Ca2+ ATPase use ATP-derived energy to maintain these ionic gradients between the intra- and extracellular compartments. During ischemia, the ATP depletion will compromise the maintenance of the gradient and a massive influx of Na+ will take place, which is accompanied by a passive influx of Cl− through the chloride channels and water molecules via aquaporin water channels. This solute and water influx in the intracellular compartment results in cell swelling and loss of cytoskeletal integrity leading to cell death [31]. In addition, N-Methyl-D-aspartate (NMDA)-receptor stimulation during glutamate excitotoxicity (see below) process further favors the entry of Na+, Cl−, and water in neurons and glia [32]. Taken that astrocytes are 20 times more numerous than neurons in the human CNS, regulation of their cell volume will therefore also play a central role in the development of edema [31]. Importantly, as long as the blood flow is disrupted, the cytotoxic edema is not leading to an increase of tissue volume, but should be rather regarded as a fluid redistribution between two compartments.
The ionic and the vasogenic edemas result from an increased permeability of the blood–spinal cord barrier. In the first phase, the depletion of ions and water from the interstitial space triggers an increased trans-endothelial ion transport, the ionic edema [33]. Thereafter, further endothelial dysfunction, in part related to cytotoxic swelling of endothelial cells, leads to the formation of permeability pores in the blood–spinal cord barrier that allows for the passage of large plasma-derived molecules, the vasogenic edema [31].
19.3.2 Early Acute Phase
The early acute phase of SCI takes place from approximately 2–48 h post-injury and is therefore the phase seen by clinicians upon admittance of the SCI patients. During this early phase, hemorrhage is still ongoing and edema and inflammation are increasing. Cells cannot maintain their homeostasis which leads to significant necrotic cell death due to cell swelling, impairment in ATP production, the disruption of organelles, and the lysis of the plasma membrane causing the release of the intracellular content in the extracellular space and the induction of local inflammatory process [34]. In this regard, Kwon and colleagues recently reported that the concentration of specific “structural” and “inflammatory” biomarkers, e.g., glial fibrillary acidic protein (GFAP) or IL-6, in the cerebrospinal fluid (CSF) of SCI patients 24 h after injury had a good prognostic value for the severity of the functional loss, i.e., American Spinal Injury Association (ASIA) impairment scale, as well as the likelihood of conversion 6 months after injury [35].
Acute cell death by necrosis following SCI is thought to be uncontrollable [36]. As a result, fulminating secondary damage processes get induced during this period: free radical production, ionic dysregulation, glutamate-mediated excitotoxicity, and immune-associated neurotoxicity. These processes provoke additional axonal injury, cell death, and the propagation of the lesion within the surrounding spared tissue.
19.3.2.1 Excitotoxicity
In experimental models of SCI, the massive release of glutamate leads within 15 min to an excessive and persistent activation of glutamate receptors causing cell death, i.e., the excitotoxicity [30, 37, 38]. When applied on uninjured spinal cords, the glutamate concentrations reached following SCI have been shown to be toxic and sufficient to induce cell death [39]. Glutamate is a central excitatory neurotransmitter in the central nervous system which binds to ionotropic receptors, such as NMDA receptor, α-amino-3-hydroxy-5-methyl-4-isoxazolepropionic acid (AMPA), and kainate receptors, as well as metabotropic receptors. The expression of these two receptor types is not restricted to neurons, and thus expression of several subtypes has been detected at the surface of astrocytes and oligodendrocytes [40–43]. As a consequence, glutamate excitotoxicity is not restricted to the gray matter tissue and has also been demonstrated to play a key role in white matter secondary damage [44].
Diverse sources are responsible for the immediate increase of extracellular glutamate following SCI. First, glutamate gets released by the direct mechanical rupture of cells and to a less extent from apoptotic and necrotic cells [45]. In the white matter on the other hand, the failure of the Na+/K+ ATPase and the collapse of Na+ and K+ gradients across axonal membranes following SCI will cause a reversal of the Na+-dependent glutamate transport and consequently an important glutamate efflux [45–47]. Mechanical cell damage and transport reversal are considered to be the major mechanisms of glutamate release following SCI [45, 48]. Within the gray matter, the imbalanced ion homeostasis in neurons leads to an increase in intracellular Ca2+ concentrations and the local release of glutamate at the synapse. Moreover, astrocytes can also discharge toxic amounts of glutamate in the extracellular milieu when their intracellular calcium levels are elevated. Finally, lipid peroxidation, for example, the formation of 4-hydroxynonenal products (see section below), impairs the capacity of astrocytes to reuptake glutamate and thereby contributes to its interstitial accumulation after injury [49, 50].
The mechanisms of excitotoxicity in the gray matter neurons are primarily associated with the ionotropic glutamate receptors and in particular the NMDA-receptors [51, 52]. Overactivation of NMDA-receptors results in an influx of Ca2+ and Na+ that contributes to the ionic gradient destabilization and leads to a necrotic or apoptotic cell death [39, 53]. Activation of the NMDA-receptor by glutamate promotes a delayed (between 1 and 7 days post-injury) cell death of neuron and glia [54]. Application of the receptor agonist (MK-801) to block the NMDA-receptor activation shortly after SCI improved the motor recovery and reduced the edema formation [55]. Similarly, the administration of a non-NMDA excitatory amino acid receptor agonist (NBQX) resulted in smaller lesion size and a better neurological status caudal to the injury site [30]. In addition, an excessive activation of Group I metabotropic glutamate receptors will upregulate expression of NMDA-receptors [56] and thereby also indirectly increased calcium influx. The influx of Ca2+ through ionotropic glutamate receptors, such as the NMDA-receptor, will lead to cell death through the activation of various secondary damage cascades including the calpain-mediated proteolytic degradation of cytoskeletal components (such as spectrin, Microtubule-associated protein 2 (MAP2), and neurofilaments), lipid peroxidation, production of reactive oxygen species (ROS), and mitochondrial respiratory failure [57–60].
Since only low amounts of AMPA-receptors can be detected on axons, the glia are considered to be the main target of glutamate toxicity in the white matter following SCI [44]. Moreover, although both astrocytes and oligodendrocytes express glutamate receptors [41, 42, 61], the latter appears to be particularly susceptible for excitotoxic cell death [61–64]. The resulting excitotoxic death of oligodendrocytes during the spreading of secondary damages provokes the loss of myelin sheath and impaired axonal conductivity.
19.3.2.2 Ionic Dysregulation
Traumatic SCI, and in particular the excessive glutamate signaling, rapidly alters the ionic membrane flux, leading to a relevant increase in the intracellular Na+ and Ca2+ concentrations and decrease in intracellular K+ levels [44, 46, 65–68]. The high intracellular Ca2+ concentration will further inhibit the mitochondrial respiration and result in an energy depletion [57]. This depletion of energy due to anoxia and decrease in ATP production causes a suppression of the Na+/K+ ATPase and the Na+/Ca2+ exchanger activities and therefore hinders the mechanism of ionic homeostasis [44, 46, 69–71].
The failure of the Na+/K+ ATPase results in a depolarization of the axonal membrane causing an even higher Na+ influx through voltage-gated Na+ channels [68]. In 1992, Stys et al. provided evidence that these conditions provoke the reversal of the Na+ – Ca2+ exchanger activity and thereby to a greater influx of Ca2+ [72]. The high density of voltage-gated Na+ channels at the nodes of Ranvier makes axons more vulnerable to excessive Na+ influx, as compared to the somatic compartment of neurons. This abnormal Na+ influx, accompanied by a passive influx of Cl− and water, is responsible for swelling of cells and edema [73]. The improper intracellular sodium–potassium balance exacerbates the cytotoxic edema [74]. High intracellular Na+ concentration activates Na+/H+ exchanger rising intra-axonal pH by proton influx, leading to axonal acidosis which further increases calcium membrane permeability [75–78]. Administration of Na+ channel blockers in animal models of SCI provided a better functional outcome and smaller lesion size, supporting the view that Na+ entry is a key player in early secondary injury mechanisms [75, 79].
Ca2+ acts as secondary messengers in diverse cellular processes. During the induction of cell death, high intracellular levels of Ca2+ activate protein kinases and phospholipases, increase the ROS generation, and cause mitochondrial dysfunction [38, 80]. Additionally, high intracellular concentrations of Ca2+ activate the calpains, which are Ca2+-dependent non-lysosomal cysteine proteases degrading cytoskeletal proteins, such as neurofilament subunits and microtubule-associated protein 2 (MAP2), which are important for axonal integrity and function [58, 81–83].
19.3.2.3 Mitochondrial Failure
Mitochondria are crucial to the cellular energy metabolism and generate ATP via the oxidative phosphorylation. Mitochondria possess a double membrane which defines four compartments: the outer mitochondrial membrane (OMM), the intermembrane space (IMS), the inner mitochondrial membrane, and the matrix. The outer mitochondrial membrane (OMM) forms a loose barrier for the intermembrane space and allows for molecules up to 5 kDa in size to pass through, for example, via the voltage-dependent anion channels (VDAC) [84]. In contrast, the inner mitochondrial membrane (IMM) is only passively permeable to oxygen, water, and carbon dioxide under physiological conditions. These differences in permeability are essential to establish and maintain a proton gradient across the inner mitochondrial membrane, which is central for the generation of ATP [85, 86].
The mitochondrial proton gradient is generated by the so-called electron transport chain (ETC). The first and largest component of the ETC, Complex I (also named NADH dehydrogenase), catalyzes the oxidation of NADH in the IMS and uses the energy liberated to pump protons in the IMS [86]. In contrast to other tissues, mitochondria in the CNS, and especially those present in neurons, contain large amounts of Complex I, which is prone to generate reactive oxygen species under physiological and pathological conditions [87]. Under physiological conditions, however, the coenzyme Q and the cytochrome c are responsible to carry the electrons along the various complexes of the ETC. This electron transport will contribute to the proton gradient in the intermembrane space and will end with the reduction of molecular oxygen within the matrix [85, 86, 88, 89].
The Complex V of the ETC, also called the ATP synthase, is responsible for the generation of ATP, the main energy carrier of the body. Complex V is embedded in the inner mitochondrial membrane and acts as a proton channel. Under physiological conditions, protons are forced through the channel, and ATP will be synthesized from this electrochemical gradient, provided that ADP and free phosphate are available. If the proton gradient is insufficient, Complex V will use ATP to pump protons back into the intermembrane space, thus consuming energy instead of generating ATP [85, 86, 88, 90].
In addition to the synthesis of ATP, mitochondria are important reservoirs responsible for buffering and maintaining the cytosolic Ca2+ levels. After SCI, however, the cytosolic Ca2+ concentrations significantly increase within the first 15–60 min and persist abnormally high for days. In the first phase, the Ca2+ influx will be largely buffered by a mitochondrial uptake, leading to an increase in ATP production [91]. The side effect of a higher activity of Complex I is however the increased production of ROS [88, 91]. Nevertheless, the mechanism by which calcium concentration influenced the generation of reactive oxygen species within the mitochondrial compartment is still debated [57, 92].
Further toxic accumulation of excessive Ca2+ within the mitochondria will trigger an unspecific permeabilization of the inner mitochondrial membrane through the opening of the mitochondrial permeability transition pores (mPTP) [90, 93]. The molecular structure of the mPTP remains unclear [94]. The opening of the mPTP enables molecules of up to 1.5 kDa to pass the inner membrane into the mitochondrial matrix. It will also lead to a severe breakdown of the proton gradient and therefore inactivates the ATP synthesis. Moreover, the attempt of Complex V to restore the proton gradient by actively pumping protons into the intermembrane space will lead to a massive ATP consumption [90, 95, 96]. Opening of the mPTP will also enable an influx of water and other molecules into the mitochondrial matrix and provoke swelling and disruption of mitochondrial membranes, resulting in a release of several proapoptotic factors within the cytosol. These factors will induce programmed cell death, i.e., apoptosis, necroptosis, and autophagy, as long as the ATP levels suffice, otherwise necrosis will occur [85, 97–100].
19.3.2.4 Free Radical Production and Lipid Peroxidation
Oxygen is particularly prone to participate in the generation of reactive free radicals, particularly in association to the mitochondrial ETC, which is the main generator of ROS and free radicals in cells. As mentioned above, mitochondria intensify the production of ROS following SCI, although the role of Ca2+ accumulation in this increase is unclear [57]. Moreover, the last step of the ETC consists in the reduction of molecular oxygen, which gives rise to a superoxide under physiological conditions. Although superoxide radicals are not very reactive per se, they can react with nitric oxide to produce peroxynitrite, a highly reactive nitrogen species (RNS). Moreover, a second reduction reaction on the superoxide will generate to peroxide, and a third reduction reaction will result in the formation of the highly reactive hydroxyl radicals [101].
In addition to the mitochondrial free radical generation, the acidosis observed after SCI promotes the release of the tightly sequestered intracellular iron stocks from the ferritin and the transferrin. The spontaneous oxidation of Fe2+ into Fe3+ will also generate superoxide radicals. Moreover, the reaction of Fe3+ with hydrogen peroxide will generate highly reactive hydroxyl radicals through the so-called Fenton reaction [102].
The toxic ROS and RNS generated under pathological conditions react on numerous targets [102]. Lipid peroxidation is likely the most deleterious ROS-associated damage occurring after SCI. Lipid peroxidation proceeds according to three distinct steps starting with the initiation in which highly reactive oxygen species react with the polyunsaturated fatty acids of the membrane and take an electron from the allylic carbons to generate a reactive lipid. This reactive lipid will interact with a superoxide radical to form a lipid peroxyl radical. The latter will react on the neighboring fatty acid and cause again the production of a reactive lipid. The propagation of this reaction will continue until the reactive lipid quenches with another radical or until no more unsaturated lipids are available. This final step, the termination, generates two toxic peroxidation products, namely, 4-hydroxynonenal (HNE) and 2-propenal [49, 102–104]. The carbonylation of amino acids is also an important free radical-associated damage responsible for the increased protein oxidation observed after traumatic SCI [105]. Similarly, RNS can nitrate the tyrosine residues to give rise to 3-nitrotyrosines (3-NT), which is a specific marker for protein damage [103].
The processes of protein oxidation and lipid peroxidation play an important role in the secondary damage cascade following SCI. For instance, lipid peroxidation will exacerbate ionic imbalance by destabilizing various membranes, e.g., cytoplasmic membrane or the endoplasmic reticulum [103]. Furthermore, lipid peroxidation contributes to the inhibition of the Na+/K+ ATPase, which worsens the intracellular accumulation of Na+ [106, 107]. The oxidative stress can also attack enzymes of the mitochondrial respiratory chain, alter DNA, etc. and results in a metabolic collapse with ensuing necrotic or apoptotic cell death [108].
19.3.2.5 Inflammation
As for other organs, lesions of the spinal cord induce strong inflammatory and immunological responses. Intriguingly, SCI evokes substantially more potent and widespread neuroinflammation than brain injury [109] and elicits a multi-organ systemic reaction. Thus, following spinal cord lesion, systemic inflammation provokes damages to the lungs, kidneys [110], and liver [111, 112]. The inflammatory processes within the injured spinal cord are complex, and their net impact, either neurotoxic or neuroprotective, often depends on their duration and timing. The inflammatory responses after SCI involve various cellular components, such as microglia, neutrophils, and macrophages, and also molecular components, such as cytokines, prostanoids, and the complement system.
Under physiological conditions, complement factors are synthesized at low levels within the CNS by astrocytes, microglia, neurons, and oligodendrocytes [113]. The complement factors are not only important effectors for the host immune response to various types of pathogens, they are also activated by tissue damage [113]. Following SCI, the complement is activated almost immediately and then increases locally within 1 day and persists thereafter chronically [113, 114]. This elevation of the complement is also detectable in the serum of SCI animal models and may become a useful biomarker in human patients [115]. The local production of complement factors by CNS-resident cells and from infiltrated inflammatory cells, as well as a transient leakiness of the blood–spinal cord barrier, results in the accumulation of these factors within the injured spinal cord [113]. Although the contribution of serum-derived complement proteins vs. local production is still unknown, the local secretion contributes to the establishment of a gradient facilitating the recruitment of phagocytic cells to the lesioned tissue for clearance of myelin debris among others. Recent studies have demonstrated that inhibition of complement system was beneficial during the acute phase of SCI (<7 days). However, its absence at later time point was associated with a larger lesion size and reduced functional recovery, as a consequence of impaired glial scar formation and increased peripheral immune cell infiltration [115].
Similarly, the cellular component of inflammation gets mobilized early after SCI. In particular, microglia react within minutes to cellular damages and to the release of ATP and nitric oxide in the extracellular milieu [29, 116, 117]. In a SCI mouse model, the induction of IL-1β expression revealed the activation of local resident microglia as soon as 5 min post-injury [29]. In human, the activation of microglia could also be detected in the earliest time point investigated, i.e., 30 min post-SCI, and the elevated secretion of IL-1β by microglia has been confirmed 5 h post-SCI [118]. Early secretion of IL-1α and IL-1β by the activated microglia during cell death-induced sterile inflammation promotes the infiltration of leukocytes toward the lesion site [29, 119]. With their progressive accumulation, infiltrating neutrophils and macrophages become the primary source of IL-1β secretion in the lesion site within 1 day [119].
The early recruitment of peripheral cells in the lesion is central to the pathophysiology of SCI, for instance, the infiltration of neutrophils, which is already extensive within the first 24 h and peaks within 3 days post-injury [120, 121]. However, neutrophils are ephemeral at the site of injury. Hence, following a maximal accumulation of neutrophils at day 3 in a rat SCI model, only half of the neutrophil population was still present 1 day later, and very few neutrophils were detectable 2 weeks post-injury [120]. Importantly, Allen et al. 2012 [122] reported that neutrophils acquire neurotoxic properties following an IL-1-mediated blood–brain transmigration. Moreover, neutrophils secrete proteases, elastase, and myeloperoxidase and release ROS and cytokines, such as TNFα, which altogether exacerbate the damages associated with the initial trauma and contribute to the recruitment of additional inflammatory cells [123, 124]. Similarly, the population of infiltrating T-lymphocytes followed a comparable kinetic as seen for the neutrophils. However, a residual population corresponding to approximately 10 % of T-lymphocytes present at 3 days was still detectable in the lesion site up to 10 weeks post-injury [120].
The size of the phagocytic cell population invading the lesion site is proportional to the extent of damages [125]. In agreement with their early activation, microglia have been reported to be the main phagocytic cells for the clearance of cellular debris during the first day following SCI [126]. Following the neutrophil wave, hematogenous macrophages immigrate in the lesion site from approximately day 3 onward and accumulate in number until day 7–10 [120, 126–128]. During this massive wave of phagocytic cell invasion, incoming macrophages become the predominant phagocytic cell type and they will sustain their phagocytic activity, whereas the microglia progressively retire from clearance activity with no or little phagocytosis detected 3 days after injury [126, 129]. In contrast to neutrophils, the macrophage population, following its accumulation peaks, only slowly resolves from the spinal cord. Hence, in the lesion, 50 % of the macrophage population present at accumulation peak was still visible 55 days after injury in a rat SCI model and 45 % at the latest time point investigated in this study (10 weeks) [120]. The lack of lymphatic drainage within the spinal cord could partly explain the longer persistence of leukocytes, although the alymphatic nature of the spinal cord following injury has been recently questioned [130].
As compared to responses following peripheral nerve injuries, a more pronounced and more sustained polarization of microglia and macrophages toward the pro-inflammatory M1 phenotype have been observed following SCI [131–133]. Moreover, the number of classically activated macrophages (M1) progressively increased over time going from 10 % CD86+ macrophages 1 week post-injury to 30 % CD86+ at 10 weeks post-injury. Recruited M1 macrophages secrete pro-inflammatory mediators and are thought to be the major source of toxic inflammatory factors following SCI [133]. Furthermore, M1 macrophages express 16-fold higher level of chondroitin sulfate proteoglycans (CSPGs) than M2-polarized cells, which could further negatively impact the regeneration potential within the spinal cord [134]. The study performed by Prüss and colleagues demonstrated that the M2-polarized macrophage population decreased from 60 to 20 % from the first to the tenth week post-injury [120]. The paucity of anti-inflammatory M2 macrophages observed after SCI might be responsible for the prolonged pro-inflammatory status, which is deleterious for the neural tissue [133]. Among others, M2-polarized macrophages secreted IL-10, a cytokine with robust anti-inflammatory activity [135]. The neuroprotective activity of IL-10 administration following SCI appears to be associated with its ability to increase the expression of anti-apoptotic genes [136, 137]. The observation that 20–40 % of the M2-polarized macrophages transplanted into an injured spinal cord switched to a M1 phenotype, whereas this does not occur in the intact spinal cord, underscores the strong M1-inducing environment prevailing following SCI [133].
Accumulated evidence shows that infiltrating neutrophils and macrophages are simultaneously involved in processes of damage and repair of the neural tissue following SCI [122, 123, 133, 138–140]. For example, blood-borne monocytes/macrophages and resident microglia recruited to the injury site contribute to the debris clearance by phagocytosis [28, 126]. The bulk of immune cells recruited on the other side secretes pro-inflammatory cytokines, e.g., TNFα, interleukins, and interferon promoting the progression of secondary injuries [141]. Moreover, although invading macrophages have been classically associated with the further destruction the neural tissue surrounding the lesion site, the phagocytic clearance capacity provided by the macrophage is even higher following peripheral nerve injury. Hence, the lower phagocytic activity seen following SCI might be paradoxically responsible for a poor regenerative response [142–145]. This apparent deleterious/beneficial duality results from the capacity of immune cells to be polarized into different phenotypical subsets with pro- and/or anti-inflammatory activities at different time points and distances from the lesion [119, 129, 138, 146].
19.3.2.6 Axonal Dieback and Wallerian Degeneration
The induction of acute axonal degeneration (AAD) is responsible for the bulk of axonal degeneration occurring during the early phase, i.e., the first 48 h post-SCI [147]. Acute axonal degeneration shares common downstream effectors of axonal degeneration, e.g., activation of the cysteine protease calpain, with the Wallerian degeneration [148, 149]. However, the process of acute axonal degeneration is characterized by the early influx of Ca2+ into the axons, as soon as 15 min after injury, through pores transiently opened by the mechanical stress [148, 149]. It has been recently shown ex vivo that clearance of extracellular Ca2+ directly after injury protects axons from acute axonal degeneration. Using in vivo calcium imaging, Williams and colleagues demonstrated the axons accumulating high levels of Ca2+ after SCI where at risk to undergo acute axonal degeneration [148]. Immunodetection of neurofilaments and electron microscopy revealed that the number of axons remaining in the dorsal column at the level of the lesion was reduced by more than half already after 1 day after compression injury in a rat model [150].
At the end of the acute axonal degeneration phase, remaining transected axons will undergo a distinct degenerative process at their proximal and distal segments. For a few hours, the axonal stumps retain their morphology and enter the so-called “latent” phase of Wallerian degeneration [149]. The end of transected axons will be capped by retraction bulbs, which are similar in morphology to growth cones. In contrast to the latter, however, the retraction bulbs have a disorganized microtubule network, which is associated with an impaired axonal regeneration capacity [151]. Stabilization of the microtubule network by the application of paclitaxel following axonal transection reduces the formation of retraction bulbs and the axonal retraction of the proximal stumps, whereas disorganizing the microtubule network of growth cones using nocodazole turns them into retraction bulbs [151].
The distal stump, which is separated from the cell soma, will disintegrate via Wallerian degeneration within the first 24–48 h post-injury in rodents or over several days in humans [152, 153]. In addition to transected axons, the signaling cascade leading to Wallerian degeneration can also be triggered following failure in axonal transport [154]. After the latent phase of Wallerian degeneration, fulgurant fragmentation along the full length of the axon takes place stochastically between the remaining axons. In the case of axonal transection, the fragmentation appears to spread anterogradely within individual axons [152, 155]. Although mechanisms underlying acute axonal and Wallerian degenerations remain largely unknown, the influx of calcium is clearly playing a pivotal role in these processes [59]. However, whereas the withdrawal of extracellular Ca2+ was shown to be protective against the acute axonal degeneration in an ex vivo model, it is rather ineffective against the Wallerian degeneration, which depends primarily on intra-axonal Ca2+ pools [59].
Wallerian degeneration shares similarities with apoptotic processes, such as being triggered by a signaling cascade and the exposition of annexin V on the plasma membrane [155]. However, the modulation of classical pro- or anti-apoptotic factors, e.g., Bax, Bak, Bcl2, etc., does not prevent or influence the course of Wallerian degeneration, thereby suggesting two distinct signaling cascades for apoptosis and Wallerian degeneration [156, 157].
19.3.2.7 Demyelination
Oligodendrocytes are particularly vulnerable following SCI and tend to undergo necrotic and apoptotic cell death. In a rat model, the number of axons demyelinated due to the acute loss of oligodendrocytes by apoptosis peaks approximately 24 h post-injury [158]. Oligodendrocytes are sensitive to excitotoxicity since they express receptors for glutamate, i.e., AMPA, kainate, and NMDA-receptors [40]. Shortly after SCI, the glutamate concentrations rise at levels toxic for oligodendrocytes provoking a Ca2+ influx and the activation of signaling cascades leading to cell death [159, 160]. In addition, oligodendrocytes are particularly prone to oxidative stress damages due to their high metabolic activity, low amounts of glutathione, high content of iron, and their abundant peroxisomes [161]. Moreover, oligodendrocytes are submitted to the free radicals released by the infiltrating neutrophils and activated microglia. Finally, immune cells invading the lesion site release pro-inflammatory cytokines, such as TNFα, IL-2, and interferon (IFN)γ, and proteases promoting apoptosis in oligodendrocytes [36, 162–164].
The apoptotic processes steadily decrease the number of oligodendrocyte over at least 3 weeks in a rodent model of SCI [165, 166]. This loss of oligodendrocytes causes a demyelination that compromises axonal function and preservation. Days, even weeks, after the initial trauma, a wave of oligodendrocytic apoptosis propagates within as much as four segments from the injury site [167, 168]. This remote apoptosis observed within the white matter is thought to result from the Wallerian degeneration which deprives the oligodendrocytes from trophic factors formerly supplied by the axons [165, 166, 169]. Since one oligodendrocyte myelinates several axons, the removal of one oligodendrocyte by apoptosis may result in demyelination of adjacent axons, otherwise considered as spared and therefore aggravates the loss of neurological functions distal to the lesion.
Polarization of microglia and macrophages into the pro-inflammatory M1 state, due to increased iron uptake and immediate increase in TNFα production, promotes apoptosis and necroptosis of neurons and oligodendrocytes [132]. Studies based on IL-1α knockout models and IL-1 receptor antagonist revealed that this cytokine plays a central key role in the induction of inflammation and apoptosis in oligodendrocytes following SCI [170]. Moreover, M1 microglia and macrophages, as well as neutrophils and astrocytes, express the pro-inflammatory cytokine TNFα [29]. At the site of injury, the mRNA expression of TNFα increases dramatically after trauma with a first wave peaking already 1 h post-injury and decreasing by 24 h [29, 171]. Modulation in mice models has shown that this early flood of TNFα is toxic for neurons and oligodendrocytes and induces their cell death [172–174].
Following injury, oligodendrocytes induce the expression of Fas-receptors, which are involved in caspase 3 and caspase 8-mediated programmed cell death [175]. Interaction of the Fas-receptor with the Fas-ligand found on the surface of activated microglia and invading lymphocytes induces apoptotic cell death [175–177]. Demjen and colleagues demonstrated that the application of Fas-ligand-neutralizing antibodies improved motor outcome and reduced apoptosis in neurons and oligodendrocytes after partial dorsal transection of the spinal cord in a mouse model [178]. Shortly after, Casha et al. reported an improved motor recovery and better survival of oligodendrocytes and axons in a Fas-deficient mouse model [176]. However, better survival of neurons was not observed in this model. Therefore, a Fas-receptor–ligand interaction might play an important role in oligodendrocytic cell death and demyelination following SCI.
19.3.3 Subacute Phase
The subacute phase (2 days to 2 weeks post-injury) is characterized by the continuation of an intensive phagocytosis of cellular debris accumulated in the lesion site [28]. In addition, during the subacute phase, the astrocytes located at the periphery of the lesion proliferate and become hypertrophic, which can be easily visualized by the detection of intermediate filament GFAP. These astrocytes initiate the formation of a glial scar physically sealing the injury site. The prominent astrocytic scarring detected in rodents has been reported to be significantly more restricted in humans [179].
19.3.3.1 Fibroglial Scar
Astrocytes are a major component of the fibroglial scar developing around the lesion site [180]. Astrocytes react quickly to SCI by changes in their gene expression, hypertrophy, and process extension [181]. The fibroglial scar consists of various cells and extracellular matrix (ECM) developing in and around the lesion. Hypertrophic astrocytes dominate in the peri-lesion, but the lesion core contains a heterogeneous mix of cells including NG2+ glia/oligodendrocyte precursor cells, meningeal and/or vascular fibroblast, pericytes, ependymal cells, and phagocytic macrophages [182].
The scar tissue forming after SCI is often referred to as the “glial scar” because of the presence of numerous astrocytes. However, the non-glial component in scars, comprising a significant amount of pericytes, and connective tissue originating from ECM deposited by fibroblasts should not be neglected [183]. Under physiological condition, there are approximately ten times more astrocytes than pericytes within the spinal cord parenchyma. However, 2 weeks following SCI in a mouse model, the lesioned segment contained twice as much pericyte-derived cells than astrocytes [184]. In this mouse model, cells originating from pericytes occupied the central regions of the scar tissue and were surrounded by astrocytes. Also, in the first days following injury, many pericytes found in the lesion site had lost contact with the blood vessels and migrated in the surrounding tissue where they secreted large amount of ECM molecules [184]. Pericytes which detached from blood vessels started to express markers characteristic for fibroblasts, such as fibronectin, and constituted the main source of scar connective tissue [184].
Disruption of the meninges (see chapter 2) by traumatic SCI is associated with infiltration of meningeal fibroblasts, which form a fibrotic scar and contribute to the inhibitory environment [185], and expression of repulsive guidance molecules [186] and can result in spinal cord tethering [187, 188]. The glial scar also stabilized the injured parenchyma by reestablishing its physical and chemical integrity, closing the blood–brain barrier, reducing the infiltration of non-CNS cells, and limiting possible infection [189]. Historically, the astrocytic scar has been regarded as the main impediment for axonal regeneration. However, recent data have demonstrated that the scar has also a pro-regenerative function [190]. Hence, depletion of the astrocytic component of the fibroglial scar has been shown to exacerbate the lesion, increase axonal retraction, and decrease the expression of pro-regenerative genes in a mouse model of SCI [191]. Moreover, the absence of scar forming astrocytes had no impact on the accumulation of CSPGs at the lesion site.
Necroptosis, a form of cell death distinct from apoptosis and necrosis, has long been a neglected process in SCI pathology. This newly recognized type of “programmed necrotic cell death” can be initiated even in the presence of classical caspase inhibitors used to block apoptosis. Evidence that necroptosis plays an important role as a mechanism of neuronal cell death after SCI was recently provided by Liu and colleagues [192]. Necrostatin-1, a specific necroptosis inhibitor, was found to be neuroprotective after SCI and decreased lesion size after SCI through a protective action on mitochondria [192–194].
19.4 Chronic Phase
The transition from the subacute to the chronic phase is characterized on the one hand by the maturation of the astrocytic scar and on the other hand by the regenerative axonal sprouting [195]. The various temporal patterns of the regenerative response of specific tracts may reflect the different regenerative mechanisms of specific neuronal populations [195]. The chronic phase begins approximately 6 months after injury and extends for the rest of the patients’ life. The process of Wallerian degeneration remains active for many years in order to remove the severed axons and the respective cell bodies [98, 196, 197]. It is considered that 1–2 years post-injury, the lesion and the associated functional deficits have for the most part stabilized.
19.4.1 Cyst Formation
The progressive enlargement of the lesion area and the formation of a cyst walled by reactive astrocytes is a hallmark of traumatic SCI [187, 198]. The process of cavitation is the result of ongoing apoptotic neuronal and oligodendroglial cell death. Moreover, recent work demonstrated that M1-polarized microglia and macrophages present in the lesion site induce reactive astrocytes to undergo necroptosis through TLR4/MyD88 signaling [199, 200]. Since astrocytes are known to support the survival of neurons and oligodendrocytes, their loss could further promote cell death of the latter.
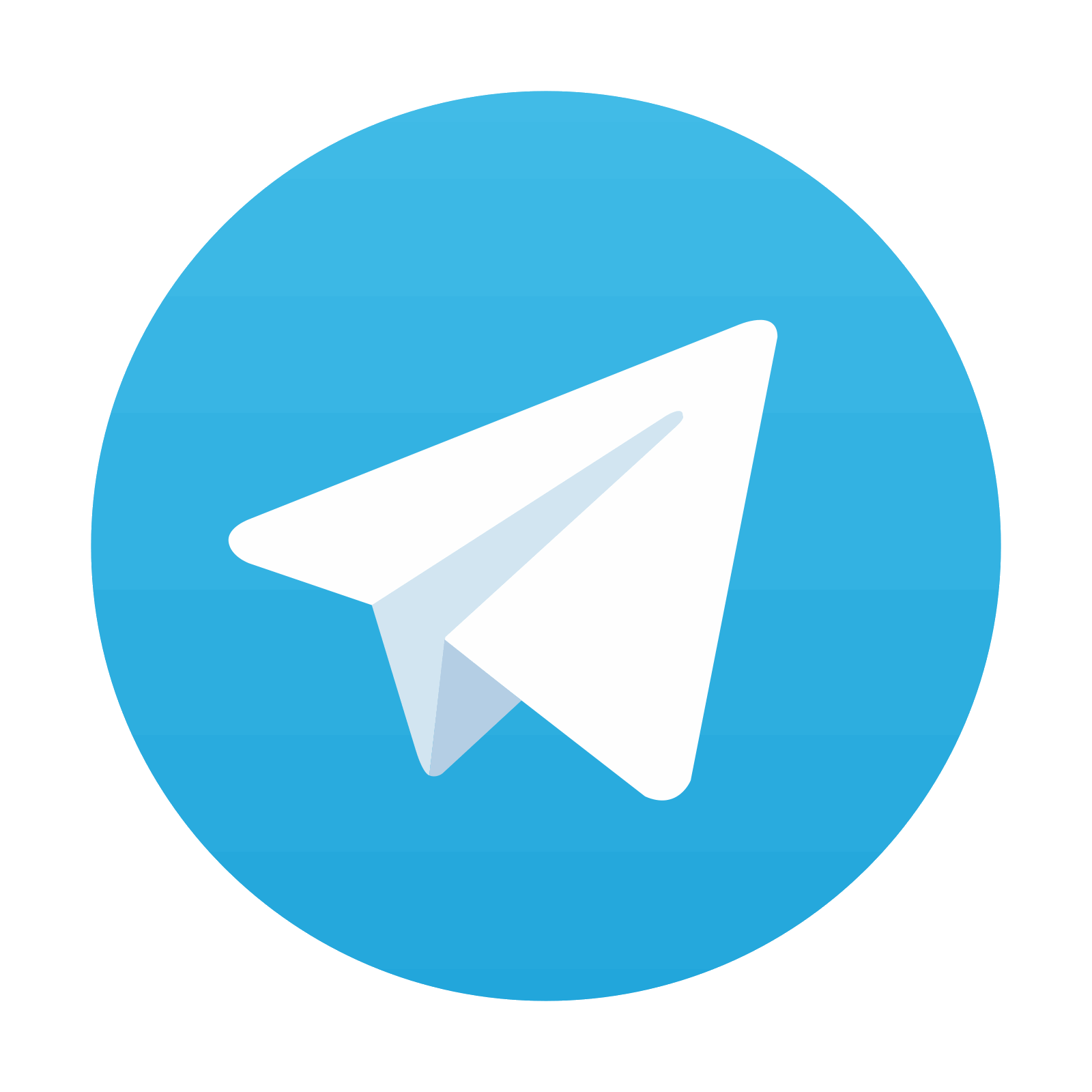
Stay updated, free articles. Join our Telegram channel
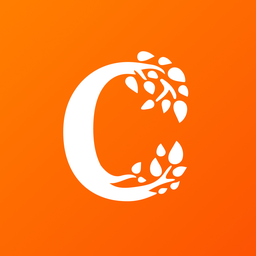
Full access? Get Clinical Tree
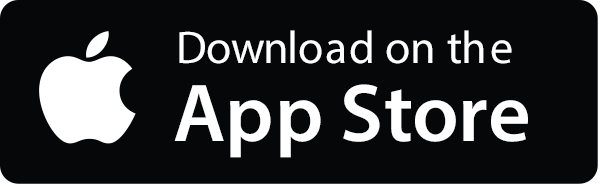
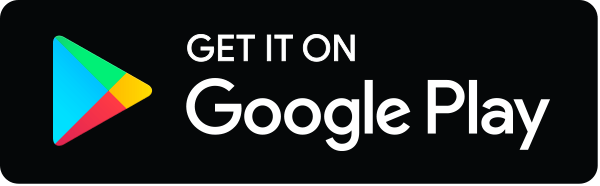