FIGURE 6-1 Schematic illustrating the relationship between state of consciousness, loss of behavioral response, and concentration of general anesthetic (GA) agent.
1. What neural event(s) underpin loss of behavioral responsiveness (LOBR) and what does this mean in terms of interoceptive awareness?
2. What brain changes occur during further increases in dose of anesthetic agent that might indicate loss of perception to sensory events (e.g., nociceptive inputs and pain)? An efficacious individualized brain-based biomarker is much needed as most anesthetics are administered based upon population responses (“average”) using nonbrain and indirect (e.g., heart and respiration rate) changes to indicate when it might be safe to start the surgical procedure without fear of pain, awareness, or movement.
3. How robust are these measures across different anesthetic agents?
4. How variable are these measures based upon other subject parameters (e.g., age, sex, presence of other drugs, brain damage)?
Early Data—Is There a Thalamocortical “Switch” in Anesthesia?
Many models have been proposed to explain “consciousness,” as discussed in the reviews recommended and this book. The “information integration” theory of consciousness states that when a percept, such as pain, is constructed then synchronous activity in distinct distributed nodes, each processing the discrete components of that percept, is necessary [10, 41]. Any breakdown of communication or connectivity between the nodes of these networks is thought to underlie loss of consciousness and will by definition impact that percept. Various studies have shown that changes in connectivity between discrete nodes occur during an anesthetic state [26, 27, 33]. An FMRI study performed by ourselves that examined the awake state and two propofol-induced sedated states (mild and moderate) in healthy subjects during concurrent auditory and nociceptive stimulation illustrates nicely how the connectivity of the thalamus and putamen to subcortical and cortical regions variably and independently changes at each of these transitional states.
When we group averaged the subjects’ brain’s responses to sensory stimulation at each of these states (awake, mild, and moderate sedation), we showed that this loss of connectivity decreased brain activity the deeper the subjects were sedated. As such, this data lends support to this theory, but the key to all these studies is our inability to know what the subjects actually perceive when sedated (as we have no behavioral measure or report). We have to infer from the brain activity map what subjects are likely perceiving based upon how these maps relate to the awake brain or a subject experiencing analgesia (if stimulus of interest is pain). Reverse inferences are fraught with assumptions—but right now it is commonly how neuroimaging studies interpret such data. Machine learning tools, discussed in the Machine-Learning Applications in phMRI section below, will undoubtedly play an important contribution to this problem going forward.
Several earlier studies (PET and FMRI) identified what was assumed to be a specific reduction in thalamic activity. This observation led to the concept of a “thalamocortical switch,” whereby thalamic suppression blocks information flow and further processing of incoming sensory stimuli within cortical regions [2, 23, 48]. While this is an attractive and intuitive construct, it is starting to be questioned within the remit of anesthesia-induced unconsciousness [3, 31]. It should be remembered that there is an order of magnitude more connections from the cortex to the thalamus than vice versa. Therefore, the reduction observed during impaired consciousness in thalamic metabolism and blood flow may be secondary to the reduced inputs from cortex or brain stem to the thalamus producing a “functional thalamic deafferentation”-like state. Alternatively, failure of information integration may be due to disruption of corticocortical communication, as recently shown using advanced analysis methods [16, 22, 34] and reduction in thalamic connectivity [1, 17, 33]. Either way, based upon current literature the verdict is that thalamic deactivation is more likely to be a consequence of impaired consciousness rather than the cause.
We should stress this seems to be the case only for pharmacologically induced or “provoked” loss of consciousness, while in physiological unconsciousness (e.g., natural sleep) the thalamus deactivates earlier than the cortex [28]. This potential dissociation between “natural” and “provoked” unconsciousness, as discussed in Chapter 5, is extremely interesting and warrants further investigation and confirmation. Certainly, anesthesia-induced altered states of consciousness appear to share some brain processes with sleep-induced unconscious states, as discussed below. Interestingly, the mechanisms underlying induction of and emergence from anesthesia-induced states of altered consciousness might be different. They might have quite separate relationships to sleep-like states, and this is the focus of current research in some laboratories such as ours.
Current Data—Multimodal Imaging and Slow Waves
Previous work using pharmacological sedation, as a model for the study of consciousness, has been largely constrained by the assumptions underlying the application of population-based pharmacokinetic models to individuals. Therefore, it is unsurprising that no individualized neurophysiological marker of perceptual loss (e.g., to pain) has been identified. The approach used in many published neuroimaging studies of consciousness or anesthesia is to deliver the anesthetic agent at specific effector-site concentrations and collect data in response to sensory stimulation for all subjects at that dose. While this has been a valuable starting point for many studies and has provided valuable data showing how the brain’s responsivity to sensory stimulation alters with increasing dose of anesthetic agent (see examples for ketamine [39] and propofol [33]), the subjects are at varying states of consciousness at each dose. The problem being that the averaged brain activation map at each dose cannot inform us about the specific link between a certain state of consciousness and the nociceptive processing relevant to pain perception. In order to achieve that understanding, we must examine each individual slowly as they transit in a continuous fashion down the curve described in Fig. 6-1. In short, we need a fundamentally different paradigm.
Due to the large and robust literature using electrophysiological measures (EEG) to explore how neural oscillations (e.g., α, δ, γ waves) alter during sleep and anesthesia [11, 35, 38], it is important to integrate these changes into any paradigm as they can inform the neuroimaging analysis. Further, we have known for a long time that under hyperpolarizing influences, including endogenous sleep drive and anesthetic drugs, cortical neurons oscillate at ~1 Hz, measurable as slow waves in the EEG. Also, it has been recently shown that in later stages of non-REM sleep, which are defined by the presence of slow waves, thalamic, and cortical responses to stimulation become less consistent [13]. Therefore, we hypothesized that the amplitude of slow-wave activity (SWA) upon reaching its maximum for an individual is the electrophysiological signature of an unresponsive yet active brain during anesthesia.
We used an ultraslow induction of propofol anesthesia (over 40 minutes induction rather than a few seconds—that is, allowing for a slow motion “video” of the brain as it transits through altered states of consciousness) and simultaneous EEG and FMRI during nociceptive and auditory stimulation—asking subjects to respond to the stimulus [36]. We showed that after LOBR each individual’s EEG SWA rises to saturation (SWAS), and remains constant despite increasing drug levels. Impressively, the magnitude of SWAS response correlated positively with their prefrontal gray matter volume. Simultaneously collected FMRI and EEG during nociceptive/painful and auditory stimulation allowed us to interrogate the FMRI responses to auditory and nociceptive stimulation during time windows guided by behavior and the EEG slow-wave patterns. Pre-LOBR there is normal activity within the brain, and this is largely sustained post-LOBR but pre-SWAS (i.e., when SWA is still low). However, during the behavioral unresponsive transition to SWAS we see a dramatic change in brain activity in response to auditory and nociceptive stimulation—in effect, perception loss and thalamocortical isolation occur. Interestingly, based upon spindle patterns, we found that an internal thalamocortical exchange persists at this stage (possibly contradicting the “thalamocortical” switch hypothesis), and an alternative more fundamental cortical network occurs that includes precuneus activation to sensory stimulation. We have established for the first time the relationship between changes in SWA and functional imaging of the brain’s response to sensory stimulation during anesthesia. From these results, we conclude that SWAS is a potential individualized biomarker for perception loss with profound implications for anesthesia and the study of altered states of consciousness [36].
This study nicely illustrates the potential for multimodal neuroimaging to reveal novel and important mechanisms related to states of consciousness and how they impact pain perception. We hope such studies provide a catalyst for other groups to work in this exciting arena.
phMRI STUDIES OF PAIN AND ANALGESIA
Stratification and Personalized Medicine
To date, most phMRI pharmacological studies have averaged the drug-induced neural response changes to a range of painful stimuli/experimental models (e.g., capsaicin model of secondary hyperalgesia and ongoing pain) within a group of approximately 20 healthy subjects to ascertain the “analgesic mechanism” [21, 25, 49, 53], or have combined FMRI with a pharmacokinetic model of the analgesic to explore the time course of its effect on pain processing [51]. As a whole, such studies show a good correlation between analgesia and reduced activity in response to these painful challenges within regions commonly found active in many pain studies (e.g., thalamus, S1, S2, anterior, mid, and posterior insula, anterior cingulate, brainstem nuclei, and prefrontal cortices). More complex mechanisms of analgesia can be identified—such as the amygdala-sensory uncoupling of connectivity during cannabinoid analgesia that correlated with the discrepancy between the change in pain intensity and unpleasantness ratings [25]. While such phMRI studies provide some validity for the neuroimaging measure relating to pain they do not confirm the specificity of these regions for pain (see below). Further, important individualized variances in network responses across these and other regions are commonly averaged out from the response, unless specific analyses are done as for the cannabinoid study. More recently, studies are being published that highlight how neuroimaging measures (functional, network, and neurochemical) at baseline, pretreatment, and posttreatment can contribute toward predicting and measuring behavioral and neuroimaging treatment responses at an individualized level. One example from our group showed that individual baseline reward system regional brain responsivity to painful stimulation predicted subsequent opioid analgesia [47]. Another study showed that posterior insula glutamate and glutamine levels and insula connectivity to other brain regions at baseline predicted subsequent pregabalin but not placebo analgesia [19]. Importantly, such studies can disambiguate drug from placebo mechanisms plus what might explain variances in the placebo response. As such, the personalized medicine and stratification goals that are much needed might be aided with the inclusion of neuroimaging. Further, they inform us about what role these brain regions contribute to pain perception and pain relief. This is important fundamental neuroscience.
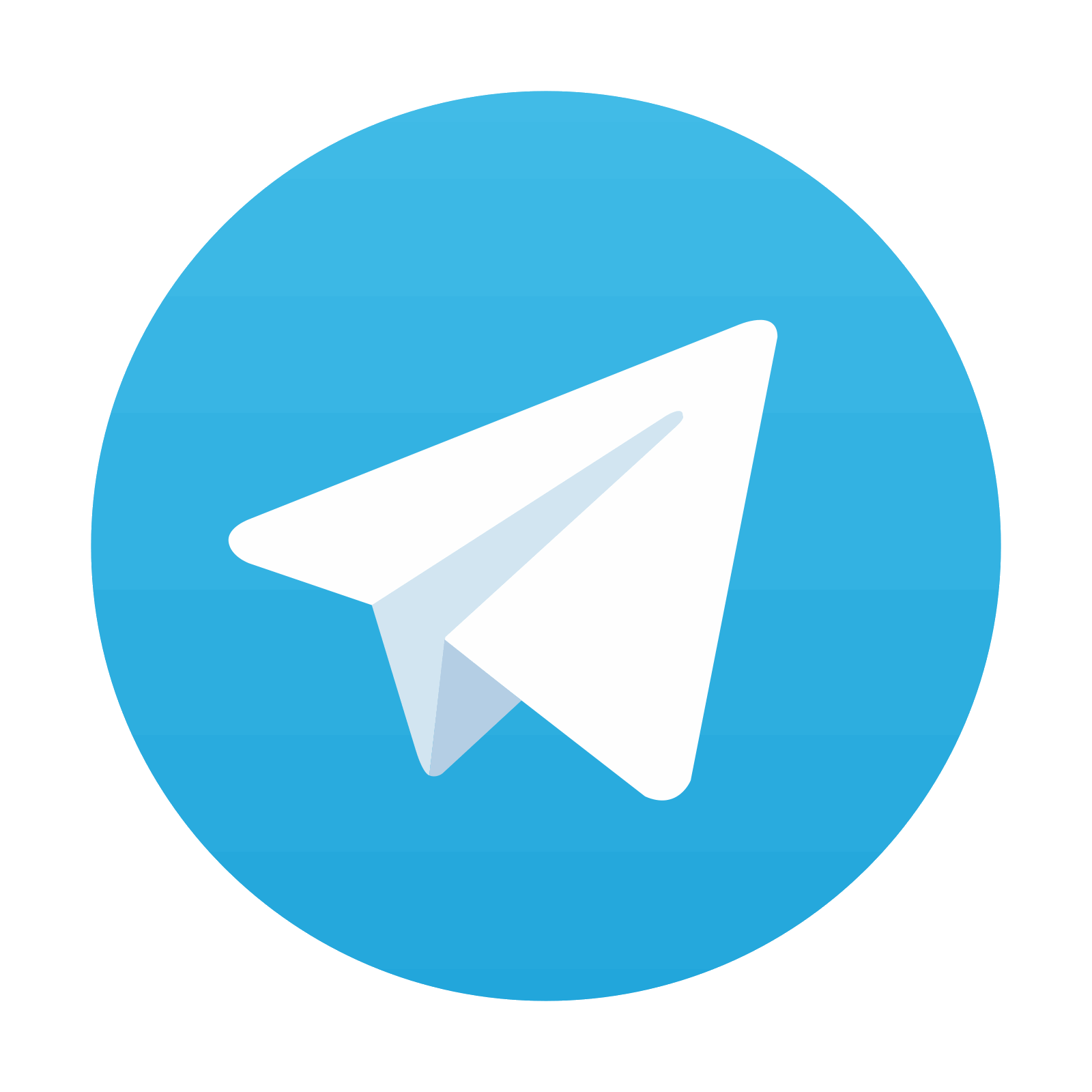
Stay updated, free articles. Join our Telegram channel
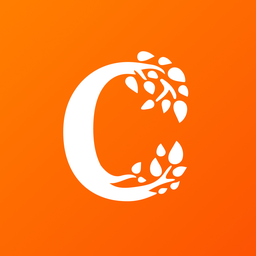
Full access? Get Clinical Tree
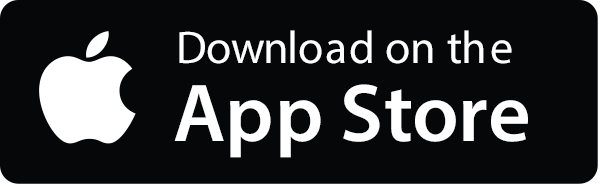
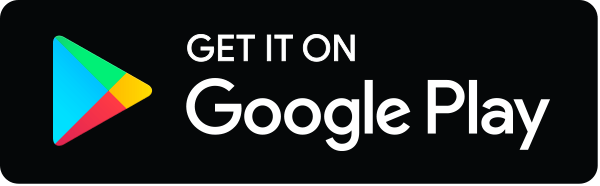