Fig. 9.1
The Fruit Fly (drosophila melanogaster)

Fig. 9.2
The Zebra Fish (danio reiro)

Fig. 9.3
The Round Worm (caenorhabditis elegans)
A model organism is a model only if, despite strong genetic similarities to humans, there is evidence that behavior meets the criteria for sleep. Drosophila quiescence does fulfill the criteria established for behavioral sleep [34, 35]. Behavioral quiescence, a stereotypic posture, elevated arousal thresholds, state reversibility with stimulation, and a homeostatic response to deprivation of rest, i.e., rest increased following the period of rest deprivation are present. This homeostatic response to deprivation is independent of at least one central clock gene, indicating that the homeostatic response is not simply a circadian response rather than a response to rest deprivation. Additional evidence for the similarity between drosophila quiescence and human sleep includes a similar response to drugs including caffeine, modafinil, and methamphetamine [36–38]. Antihistamines increase quiescent periods in drosophila similar to drowsiness in humans [35]. Finally, there are age-related changes in sleep similar to the changes in sleep amounts across the human life span [35, 39].
Drosophila is the organism which has arguably received the most attention as a model system for the genetic and molecular study of sleep. However, zebrafish (D. rerio) also offer similar biological and behavioral properties with respect to sleep for consideration as a model organism [40, 41]. Another proposed model organism is the round worm (C. elegans). During lethargus, a quiescent behavioral state which occurs after each of four molts, the behavioral criteria for sleep, including a homeostatic response to rest deprivation and elevated arousal thresholds, are present [42, 43]. There is genetic and molecular conservation between drosophila and C. elegans [44, 45]. A recent study also provides evidence that quiescence during molts in another worm, the tobacco hornworm (Manduca sexta), meets the behavioral criteria for sleep [46]. Thus, these model organisms with their practical and experimental advantages may have the potential to observe, and perhaps unlock, the underlying mechanisms controlling the expression of sleep.
Optimal Sleep and Mortality
The ill effects of insufficient sleep may be witnessed on some of the principal organic functions, but it is the brain and nervous system that suffer chiefly in the first instance. The consequences of a too protracted vigil are too well known to be mistaken, and many a person is suffering, unconscious of the cause, from the habit of irregular and insufficient sleep.
William A. Hammond, 1866 [47].
The consequences of insufficient sleep have long been a subject of debate and discussion. Still today, some of the most common questions asked of sleep clinicians revolve around sleep quality and sleep amounts: How many hours of sleep per night are necessary for good health, is a lack of sleep harmful, and will inadequate amounts of sleep or poor quality sleep affect longevity? As we shall see, there are data to suggest that too little or too much sleep may impact health and life span in humans. For example, persistent, but not intermittent, self-reported insomnia is associated with an increased risk of all-cause mortality over a 20-year follow-up period, suggesting that decreased sleep amounts negatively impact life span [48]. In contrast, prolonged sleep amounts have been associated with an increased risk of fatal and nonfatal stroke [49], conversely suggesting that increased sleep amounts negatively impact health. Although studies of sleep in nonhuman organisms reveal significant variations in sleep amounts, there are no data available which shed light on the impact of these variations in promoting species survival and longevity. As we shall see, however, there are examples of “natural” sleeplessness in the animal world, and most importantly the genetic manipulation of model organisms has advanced our knowledge about the mechanisms controlling sleeplessness.
One approach to addressing the question of whether there are optimum amounts of sleep associated with a long life span would be to examine the relationship between sleep amounts and mortality in large populations. A systematic relationship between human sleep and mortality was first described over 30 years ago [50], and since that time a body of epidemiological literature has accumulated which suggests fairly consistently that short and, somewhat inconsistently, that long sleep amounts are associated with increased mortality as well as with diseases such as obesity, diabetes, hypertension, and cardiovascular disease [51–55]. Typically, the best survival curves are associated with about 7 h of sleep per night. Shorter sleep times are variably defined in these studies as 4–6 h per night and long sleep times as more than 8–9 h per night, raising the question of whether the common recommendation of a minimum of 8 h of sleep per night is appropriate [56]. Of note is that in virtually all epidemiological studies, sleep duration was assessed by subjective estimates and not overnight sleep studies utilizing polysomnography, suggesting the possibility of subjective over- and underestimates of sleep duration which could bias results. Also, it cannot be determined in these epidemiological studies whether short and long sleep durations are the direct cause of increased mortality or whether short and long sleep durations are the product of an underlying intervening condition which in turn is responsible for these associations with mortality.
The most extensive and rigorous review of the literature addressing the question of optimal sleep time was recently performed by a panel of sleep experts assembled by the American Academy of Sleep Medicine and the Sleep Research Society [57]. The consensus of the panel was that less than 6 h of sleep is inadequate to support optimal health, but no consensus could be reached about the effects of 9 h or more on optimal health. The final consensus was that 7 h was the minimum amount of daily sleep necessary to support optimal health. However, no consensus could be reached about an upper maximum threshold of sleep for optimal health. This is not surprising. A careful review of the epidemiological studies supporting a relationship between sleep duration and mortality raises the issue of, for example, how variable methodologies for determining total sleep time may weaken the conclusions which can be drawn from the U-shaped curve characteristic of the relationship between sleep duration and mortality [58]. Also of interest in these considerations of mortality and sleep duration is the demonstration of a dose–response relationship between hypnotic sleep medications typically used to treat short sleep and an increased mortality risk as well as an increased cancer risk for the most frequent users of hypnotics [59]. These findings suggest that intervening variables, inconsistently assessed in epidemiological studies, may contribute substantially to the association between sleep duration and mortality.
Human epidemiological studies, although inviting speculation about the predictive value of sleep amounts in determining life span, do not reveal the biological mechanisms responsible for this relationship. There are age-related changes in drosophila sleep similar to the sleep fragmentation and decreased sleep amounts observed in aging humans, suggesting that drosophila may shed light on the mechanisms mediating sleep and longevity in humans [39]. Several genetically engineered drosophila mutants, including Minisleep (mns), Hyperkinetic (Hk), Sleepless (sss), and Insomniac (inc), have been developed which have markedly reduced sleep amounts in comparison with wild-type flies [60–62]. Life span in these short sleeping flies is reduced, suggesting that sleep duration has a direct relationship to longevity. Of note is that waking behavior was not impaired in mutant strains. These mutations are mediated by Shaker, a gene which encodes for the voltage dependent potassium channel. Another short sleeping mutant, fumin (fmn), which has a mutation in the dopamine transporter gene (DAT), does not show a reduced life span nor do these flies exhibit a homeostatic response to sleep deprivation [63]. There is substantial evidence from a number of studies to indicate that dopaminergic systems have a major role in the expression of arousal and sleep [36, 64]. Mutations of the amnesiac (amn) gene, which has a role in the adenylate cyclase/cAMP signaling pathway, are associated with fragmented sleep and, similar to fmn, are not associated with a rebound in quiescence following deprivation [65]. Of note is that a high-calorie diet in fmn mutants results in further reductions in sleep amounts and reduced longevity, suggesting that accelerated aging and shortened sleep are impacted by increased caloric intake [66]. Also time-restricted feeding with food access limited to 12 h per day in wild-type flies, although not changing caloric intake, is associated with improvements in sleep and ameliorates an age-related cardiac decline [67]. These studies indicate that there are a number of different factors which affect the expression of sleep. Also of interest are mutations in genes which would not seem to play a role in sleep expression such as the fragile X mental retardation gene (Fmr1). Overexpression of drosophila Fmr1 results in shortened sleep, whereas the loss of Fmr1 is associated with significantly longer sleep amounts as compared to control flies. In neither mutant was there a homeostatic response to sleep deprivation, and of particular interest relevant to the human epidemiological studies is that life span is reduced in both the short and long sleeping Fmr1 mutants [68]. Finally, it is most likely that there is not a single dedicated “sleep gene,” but that other genes more broadly controlling cellular and neuronal functions also control the expression of sleep. An example is the demonstration that decreasing cyclin A, a protein which regulates the progression of cells through the cell cycle, results in decreased total sleep and a decrease in the homeostatic response to sleep deprivation [69]. More recently, a sleep regulating protein redeye (rye) which interacts with sss has been identified in short sleeping mutants and after sleep deprivation in wild-type drosophila [70].
The cellular mechanisms controlling sleep in humans are virtually unknown. The complaint of insomnia, difficulty falling and staying asleep typically with resulting daytime fatigue, affects approximately 30 % of the population in varying degrees of severity [71]. Not only physiological factors, but the contribution of psychological factors to this disorder, make it a daunting task to untangle the mechanisms which are involved in the expression of human sleeplessness. There are two disorders characterized by shortened sleep which have been identified in this category of sleep difficulties and which have a known genetic basis. The first is advanced sleep phase syndrome in which affected family members have a mutation of the hPER2 clock gene involved in controlling the timing of sleep. Although the sleep cycle is regular in affected family members, there is a four hour advance of sleep, temperature, and melatonin rhythms in affected as compared to unaffected family members [72]. A second disorder of sleeplessness with a known genetic basis is fatal familial insomnia. This disorder is a rare, inherited, progressive neurodegenerative disease in which there is a progressive inability to sleep eventually culminating in total sleeplessness and death [73]. Autopsy findings reveal selective bilateral neuronal loss and reactive gliosis of the anterior and dorsomedial thalamic nuclei with an accumulation of prion protein. In affected individuals, there is a single mutation on the prion protein gene PRNP at position 178 combined with a mutation at position 129 [74]. Although these are two very specific instances of sleep difficulty, there is, however, evidence to suggest that short sleepers do carry a gene with a specific DEC2 (also known as BhLHE41) mutation. This mutation was identified in a family with two individuals who had lifelong short sleep averaging 6.25 h per day as compared to noncarrier family members who averaged 8.06 h per day [75]. Subsequent work has identified other mutations of BHLHE41 associated with decreased total sleep time and with fewer average lapses in performance on a psychomotor vigilance task, suggesting resistance to the effects of sleep deprivation [76].
Despite this large body of literature which suggests that sleep and longevity are related, there continues to be no clear answer to the question of whether there is an optimal amount of sleep which can promote maximum longevity in humans. Additionally, understanding the genetic and cellular mechanisms responsible for human sleeplessness is clearly in its infancy. There are, of course, no formulas to translate the equivalency of drosophila sleep minutes which can be manipulated by these mechanisms into human sleep hours. As previously noted, at least some of the ambiguity in human studies may be related to subtle differences in survey questioning about sleep amounts which result in subjective under- or overestimation of sleep time [58].
There are also variables identified from drosophila studies which may interact with genetics to potentially affect sleep with respect to longevity including social enrichment or isolation, environmental conditions, diet, methods of evaluating quiescence, and climates and altitudes [77–82]. There is also recent evidence that variations in population density during normal drosophila larval development affect sleep duration in adults, but not in amn mutants, suggesting lifelong cellular changes in sleep controlling mechanisms dating from infancy as the result of environmental exposures [83]. Although not as intensively studied as drosophila, other mammals and nonmammals also demonstrate that environment and social experience may affect the expression of sleep. For instance, sleep in honey bees is increased by exposure to the bee colony environment in comparison with isolated bees [84]. Electrophysiological recordings of unrestrained sloths in the rain forest reveal that total sleep time is substantially less than under laboratory conditions [85]. The threat of predation and social status may also play a significant role in determining sleep amounts in mammals [86–88]. In reptiles, young caimans recorded in a colony exhibited differing electrophysiology from caiman recorded in isolation, suggesting that these differences in environment and socialization may have affected the expression of sleep [17, 22].
The most striking example of the evolutionary effects of habitat upon sleeplessness is illustrated by studies in the Mexican cavefish (Astyanax mexicanus) [89, 90]. Surface- and cave-dwelling populations of these fish differ remarkably in daily sleep amounts with surface fish averaging over 800 min and three different cave-dwelling populations averaging 110–250 min per day. Blockade of B-adrenergic receptors with propranolol produced dose-dependent increases in cave fish sleep without any effect at any dose on sleep of surface-dwelling fish. Adrenergic antagonists did not affect sleep in surface dwellers, but cavefish sleep increased significantly in response to the B1 antagonist atenolol. The number of catecholamine neurons is conserved in cavefish as opposed to surface-dwelling fish, suggesting that an increase in the adrenergic arousal system in cavefish as compared to surface dwellers has occurred during evolution. Other recent fish studies have examined circadian rhythmicity in aging killifish (Nothobranchius) as well as the induction of quiescence by melatonin in the three spot wrasse (Halichoeres trimaculatus) [91, 92].
In summary, much of the appeal of the findings from human epidemiological studies resides in the simplicity of the U-shaped curve which suggests that the relationship between longevity and sleep is straightforward. Both short sleep and long sleep are associated with increased mortality. However, as we have seen from the animal literature, these data are fraught with many potentially uncontrolled and confounding genetic, environmental, and ecological factors which have the potential to alter this relationship. An unambiguous answer to the question of how much sleep and under what conditions are necessary for optimal longevity remains unanswered.
Pharmacological Development
A significant advantage of utilizing model organisms to explore the molecular basis of sleep lies in their well-studied genome which is amenable to precise manipulation. Another potentially productive area in which these model organisms may be of substantial benefit is in the area of developing new and focused pharmacological treatments for human diseases, including sleep disorders [30]. For example, approximately 70 % of human genes have at least one zebrafish orthologue, and as a result, zebrafish have been extensively used to develop human disease models [28, 93]. The correspondences between the human genome and the genomes of model systems suggest that model organisms could be instrumental in developing in vivo drug treatments at a molecular level for modifying or treating human sleep disorders. Zebrafish are sensitive to major classes of drugs including anxiolytics, hypnotics, and stimulant among others which could potentially be used to treat disordered sleep [33, 40]. In addition, the cost of screening pharmacologically active compounds is substantially less expensive in an organism such as the zebrafish so that an increased number of compounds can be economically evaluated. New effects and mechanisms of drug action on waking and quiescence produced by similar major neurotransmitter pathways in both zebrafish and mammals, and identification of poorly understood compounds can be rapidly assessed.
Cross-translational studies between model organisms and humans also open new avenues for the discovery of waking and sleep biomarkers which may have practical application. One example is the discovery of salivary amylase as a biomarker for sleepiness and sleep drive in both drosophila and humans utilizing cross-translational studies [38, 94]. The potential applications of such a “sleepiness marker” are far reaching. For example, multiple sleep latency testing (MSLT), a series of daytime nap tests spaced throughout the day according to a standard protocol, is currently the standard electrophysiological assessment tool for objectively evaluating a patient’s subjective complaint of sleepiness. The maintenance of wakefulness test (MWT) is used in a similar protocol to evaluate daytime alertness [95]. With further elaboration of the salivary amylase findings, convenient, rapid, cost-effective alternatives to the MSLT and MWT could potentially be developed for objective assessment of sleepiness and alertness in settings where polysomnography is unavailable. Another practical application of these findings may be the assessment of drowsy drivers similar to breathalyzer assessments of alcohol consumption or the assessment of persons such as air traffic controllers, bus drivers, or train conductors whose occupations require a high degree of alertness to ensure public safety.
The utilization of model organisms for development of effective pharmacological treatments and sleep-related biomarkers is still in development. However, as knowledge about sleep mechanisms continues on a rapid, steep trajectory, it is not unreasonable to anticipate that the practical application of this knowledge will also follow.
Sleep Disorders
Narcolepsy
The mechanisms underlying the expression of narcolepsy were virtually completely unknown until the serendipitous discovery by Dr. William Dement of a dog with what appeared to be cataplectic attacks similar to the cataplectic attacks demonstrated by human narcoleptic subjects [96]. Since that time, understanding the etiology of narcolepsy has arguably been primarily the result of discoveries in animal research [97, 98]. The cardinal symptoms of narcolepsy include excessive daytime sleepiness, hypnagogic hallucinations, sleep paralysis, and cataplexy, a sudden loss of muscle tone with strong emotions. Electrophysiologically, narcolepsy is diagnosed by the rapid onset of REM sleep, typically with a latency of less than 15 min as compared to the 60- to approximately 90-min latency to the onset of REM sleep in normal subjects. Narcolepsy, in conjunction with a history of clinical symptoms, is diagnosed by the rapid onset of REM sleep during a protocol of daytime nap testing [95]. Based on epidemiological studies in several countries, the prevalence of narcolepsy has been estimated at between 25 and 50 per 100,000 persons [99]. Of all the sleep disorders, the genetics, neuropharmacology, and molecular mechanisms of narcolepsy appear to be the best understood [100]. The discovery of orexin (hypocretin) deficiency resulting from the loss of orexigenic neurons in narcoleptic dogs, mice, and humans and the close association of the human leukocyte antigen DQB1*0602 and DQA1*0102 in almost all narcoleptics has led to the conclusion that narcolepsy is an autoimmune disease [101]. However, the mechanisms by which orexin is depleted are unknown. Potential environmental triggers for the development of narcolepsy which have been identified include upper airway infections and the H1N1 flu vaccine in genetically susceptible individuals [102, 103].
Gene therapy clinical trials are now being performed for a variety of human diseases including cancer, cardiovascular disease, Parkinson’s disease, and cystic fibrosis [104]. Hypothalamic gene replacement therapy in orexin-deficient mice improves sleep quality and the timing of REM sleep, but does not improve cataplexy [105]. Conversely, gene transfer into the zona incerta in orexin-deficient mice improves cataplexy, but not sleep fragmentation [106]. There are no data on gene replacement in narcoleptic humans, but the findings in mice suggest that this approach may be a promising one. As the result of this research in narcolepsy, a new sleeping medication, suvorexant, has been developed as a treatment for insomnia. Suvorexant is a dual orexin 1 and orexin 2 receptor antagonist which dose dependently enhances sleep in humans [107, 108]. Suvorexant was approved for human use by the Food and Drug Administration in August, 2014. Of interest is a detailed account of suvorexant’s approval process which appeared in the popular press [109].
A major stumbling block in the use of model organisms to study narcolepsy is the absence of REM sleep. Birds are the only nonmammalian organism to display convincingly behavioral and electrophysiological signs of REM, but avian REM sleep bouts are brief, lasting only a few seconds. Could it be possible that REM sleep is present in drosophila or zebrafish and that it has simply been missed? This seems unlikely particularly in light of a recent detailed video analysis of zebrafish eye movements and respiration during sleep which did not uncover any credible evidence for the presence of REM [110]. Additionally, the limited behavioral repertoire of model organisms precludes their usefulness in evaluating, for example, cataplexy or sleep paralysis which are major signs of narcolepsy. The drosophila brain does not contain orexin. However, zebrafish have a distribution of orexin expressed in the brain in a fashion similar to that of mammals [111]. Overexpression of orexin in zebrafish results in elevated motor activity, decreases in arousal thresholds, and shortened, disturbed sleep in the dark [111, 112]. During the course of evaluating zebrafish hypocretin receptor mutants, Yokogawa et al. [112] made several interesting observations with respect to sleep. Normal adult zebrafish maintained under constant light conditions have an almost complete suppression of sleep. A homeostatic rebound response to this sleep deprivation was not observed, and a progressive return to sleep occurred over the course of one to two weeks. Following sleep deprivation in response to electrical stimulation, there was no homeostatic response when animals were released into light, but a homeostatic rebound occurred with release into darkness. Additionally, exposure to light during the last 6 h of the biological night produced a marked suppression of sleep without a homeostatic rebound with release into darkness.
Although the orexigenic substrate of the zebrafish brain parallels that of the mammalian brain, the zebrafish is not a particularly enlightening model organism to elaborate upon mechanisms controlling narcolepsy. None of the model organisms studied to date would appear to serve as a model for narcolepsy. Furthermore, the unusual response of zebrafish sleep to light with sleep suppression and to the absence of a homeostatic response following sleep deprivation suggests that there are components of zebrafish sleep which should not be considered as a model substrate for sleep disorders.
Sleep Apnea
The cardinal symptoms of obstructive sleep apnea (OSA) are well known and unmistakable in their presentation: loud snoring and pauses in respiration terminated by explosive snores, breath holding episodes witnessed by a bed partner, and excessive daytime sleepiness resulting from the arousals terminating often hundreds of respiratory pauses during sleep. Severe OSA is a risk factor for arterial hypertension, heart failure, stroke, pulmonary hypertension, and maternal morbidity [113, 114]. A familial component has been described in several studies [115–117]. There are two reports of a naturally occurring model of sleep apnea in the English bulldog which has a crowded upper airway anatomy similar to sleep apnea patients [118] and in obese miniature pigs [119]. Nonmammalian species have not been observed to have sleep apnea, and model organisms have not been developed for experimental evaluation of this disorder.
The experimental research on sleep apnea has been focused on the induction of sleep apnea in dogs, rats, and mice by artificially occluding the airway and exposure to repetitive hypoxemia [120–123]. The cardiovascular and neurochemical consequences of sleep apnea have been described in these experimental models, but the genetic and cellular components of sleep apnea are not well understood. OSA is a complex disease process with multiple interactive factors including age, weight, gender, arterial and pulmonary hypertension, cardiovascular disease, and metabolic disorders. It is almost certainly the case that there are multiple cellular and genetic components contributing to the expression of OSA. A gene encoding the allele APOEe4 which is essential for cholesterol metabolism and transport has been associated with OSA in adults and children. However, a meta-analysis of studies reporting an association between APOE and sleep apnea concluded that the association is weak [124]. Another meta-analysis of OSA genetic association studies revealed that TNFA rs1800629, which may also be associated with heart disease and heart failure as well as chronic obstructive pulmonary disease, was significantly associated with OSA [125]. These authors concluded that studies examining OSA genetics did not typically provide robust evidence. Thus, the complexity of OSA presents significant challenges in understanding the genetics of this order, and at least at the present time, a model organism with a well-known genome combined with the symptoms of OSA does not appear to be on the horizon.
Restless Legs Syndrome (RLS)
Clinical symptoms of RLS include restless, creeping, crawling, uncomfortable sensations in the legs during sedentary activities with a worsening of these sensations beginning in the evening. They are accompanied by an irresistible urge to move the legs for temporary relief. Typically, there is a worsening of the sensations at sleep onset which results in substantial difficulty falling asleep. Key factors in the expression of RLS are iron deficiency and the involvement of the dopaminergic system in regulating iron metabolism, although the mechanism of this relationship is not clearly understood. Currently, dopamine agonists, such as ropinirole and pramipexole, which enhance brain dopamine, are the treatments of choice for RLS [126]. Prevalence, depending upon the complexity of survey questions, ranges between 9.4 and 15 % querying RLS as a single symptom. This range changes somewhat to 3.9–14.3 % utilizing the criteria of the International Restless Legs Syndrome Study Group [127, 128]. Inclusion of stricter diagnostic criteria such as the frequency and severity of symptoms results in decreased estimates of prevalence.
Genome-wide association studies (GWAS) have opened a new window onto the genetic underpinnings of human RLS. GWAS studies in both European and US populations have identified genomic loci which are associated with restless legs syndrome including MEIS1, BTBD9, and MAP2K5/LBXCOR1 on chromosomes 2p, 6p, and 15q [129–131]. Animal models have been proposed for the study of restless legs syndrome. Mice and rats with lesions of the A-ll dopaminergic nucleus which projects to the spinal cord have demonstrated an increase in locomotor activity [132, 133]. Spontaneously hypertensive rats exhibit increased motor activity, suggesting a possible model for the study of RLS [134]. One study in C. elegans demonstrated that the MEIS1 worm orthologue increased ferritin expression and human cells cultured in iron-deficient conditions revealed decreased MEIS1 expression, lending further support to the role of these genes in iron metabolism [135].
Animal studies are typically the catalyst for human research. However, the discovery of these potential RLS loci via human GWAS provided a reverse “human to animal” stimulus for the development of a drosophila RLS model [136]. As described above, the diagnosis of RLS in humans is accompanied by reports of an irresistible urge to move the legs for relief of discomfort. Of course, these sensations cannot be communicated by drosophila, but movement can be operationally used to infer the presence of RLS. Genetic alteration of the fly homologue dBTBD9 which corresponds to human BTBD9 resulted in fragmented sleep characterized by a decrease in the duration of sleep bouts and an increase in the number of sleep bouts and waking after sleep onset, suggesting the sleep fragmentation of RLS patients. However, sleep duration in flies per 24-h period was not different between mutants and controls. Although flight and negative geotaxis were normal in mutants, when confined to a restricted space flies were hyperlocomotive, reminiscent of the movements experienced by RLS patients during the forced immobility test (FIT) [137]. Uninterrupted bouts of walking were also longer in mutants. Comparing dopamine levels in mutants and controls, mutants revealed a 50 % reduction in dopamine, suggesting a relationship between alterations between BTBD9 and maintenance of normal dopamine levels. Also of note is that treatment with pramipexole, a dopamine agonist, restored sleep consolidation in mutants to control levels, again suggesting a significant role for dopamine in the expression of RLS. dBTBD9 is also implicated in the regulation of iron metabolism and ferritin homeostasis.
These genetic explorations into the expression of RLS in a model system provide evidence of the complex relationship between specific genes and the role of dopamine in regulating ferritin levels. Ideally, with further studies more effective treatments for RLS will be discovered.
Unusual Sleep Disorders
There is a group of unusual sleep disorders, the parasomnias, in which the distinction between waking and sleep becomes blurred and waking behavior appears to intrude into sleep. Included in this group of disorders are confusional arousals, REM behavior disorder (RBD), sleep walking, and sleep-related eating disorders [138]. Complex vigorous motor activity which can become violent in the case of RBD and loud vocalizations may accompany these disorders. Not a great deal is known about the prevalence of parasomnias, but sleep walking, for example, is relatively common with a lifetime prevalence estimated at 29.2 % [139]. RBD is rarer with a prevalence of 2.01 % and subclinical RBD estimated at 4.95 % in a Korean elderly population [140]. Due to the complexity of behavior in parasomnias, no animal models have been developed to study these disorders, and not surprisingly no naturally occurring animal models of parasomnias have been identified. However, early studies in cats revealed “dream enacting” behavior following pontine tegmental brainstem lesions, reminiscent of the vocalizations and motor movements in RBD [141, 142].
Can sleep and wakefulness be present in the same brain at the same time? This possibility seems counterintuitive to our normal experience since we typically dichotomize states of alertness as being either waking or sleep and the transition between them as drowsiness, often euphemistically described as being half awake or half asleep. The parasomnias suggest that the comingling of sleep and waking in the same neural substrate is possible, and insights from animal studies are of benefit in shedding light on this issue.
There are a number of examples in the animal literature in which “nonquiescent” or literally “motorically active” sleep is present, suggesting that sleep can be compatible with behavior typically present during waking. For example, nocturnal “sleep swimming” fish inhabiting coral reefs in the Red Sea vigorously move the dorsal, pectoral, and caudal fins in fixed body positions at a frequency of strokes approximately twice the rate with daytime swimming outside the coral reef [143]. This behavior may function to aerate the reef and assure healthy corals. Captive dolphin and killer whale neonates and their mothers remain continuously active and do not exhibit signs of behavioral sleep for several months postpartum with gradually increasing periods of behavioral quiescence which eventually return to normal amounts, but do not exceed normal amounts, of behavioral sleep [144, 145]. Dolphins are able to maintain continuous vigilance with accurate echolocation for a testing period of up to 15 days [146]. In the laboratory birds demonstrate marked decreases in EEG defined sleep during the migration season, and data from freely moving swifts equipped with data loggers during a 200-day nonstop flight suggest that sleep occurs during periods of decreased activity during flight [147–149].
There is the possibility that these animals are unique and simply do not sleep. However, this would be contrary to findings from virtually all other living organisms. Alternatively, a variant of sleep may be present which coexists with waking and which allows for sustained vigilance in the presence of physiological sleep. In fact, there is a unique form of sleep, unihemispheric sleep, a state in which one hemisphere of the brain exhibits waking EEG activity simultaneously with sleeping EEG activity in the other hemisphere. Unihemispheric sleep has been recorded in dolphins [150], whales [151], fur seals [152], and birds [9]. Only NREM sleep has been observed in whales and dolphins, whereas in birds and seals REM sleep is present. Of interest is a recent demonstration of decreased orexin bouton density in the cerebral cortex of a porpoise and a whale compared to ten mammalian species [153]. The presence of unihemispheric sleep offers the significant advantages of an ability for part of the brain to sleep during long-distance migration while maintaining sentinel functions in monitoring the environment [148, 154].
Unihemispheric sleep is clearly an unusual form of physiological sleep. However, there are recent human electrophysiological studies utilizing scalp, intracerebral EEG, and unit recordings which suggest the possibility that there are also coexisting regional differences in human sleep and waking. For example, detailed sleep electrophysiological studies have demonstrated that 85 and 75.8 % of sleep spindles and slow waves, respectively, have been detected in less than half of brain recording sites [155]. This regionality of sleep waveforms, along with the demonstration of unihemispheric sleep in animals, suggests that parasomnias such as sleep walking or RBD may be a manifestation of simultaneously occurring waking and sleep processes. There is also further evidence from intracerebral recordings that motor cortex activation lasting from 5 to more than 60 s can occur simultaneously with an increase in slow wave activity in the dorsolateral prefrontal cortex [156]. Also relevant in this regard is the finding that human sleep spindles recorded in the hippocampus precede the sleep spindles and K complexes characteristic of Stage 2 sleep recorded from neocortical scalp electrodes [157]. Similarly in cats, increases in ventral hippocampus spikes precede the onset of NREM sleep, also suggesting that sleep processes may begin in different brain regions at different times in both animals and humans, strengthening the similarities between these electrophysiological processes [25]. Studies in both the echidna, a primitive egg laying mammal considered the basal stock of living mammals, and the ostrich, a basal bird, demonstrate that elements of both NREM and REM sleep may be simultaneously present during behavioral sleep [158, 159]. Further illustrating the simultaneous presence of different physiological states in humans are data from a single subject with stereotaxically implanted electrodes who experienced confusional nocturnal arousals [160]. During these episodes, there was localized activation of the motor, cingulate, insular, temporopolar, and amygdalar cortices in the presence of slow waves recorded from the frontal and parietal dorsolateral cortices and in the presence of spindles recorded from the hippocampal cortex.
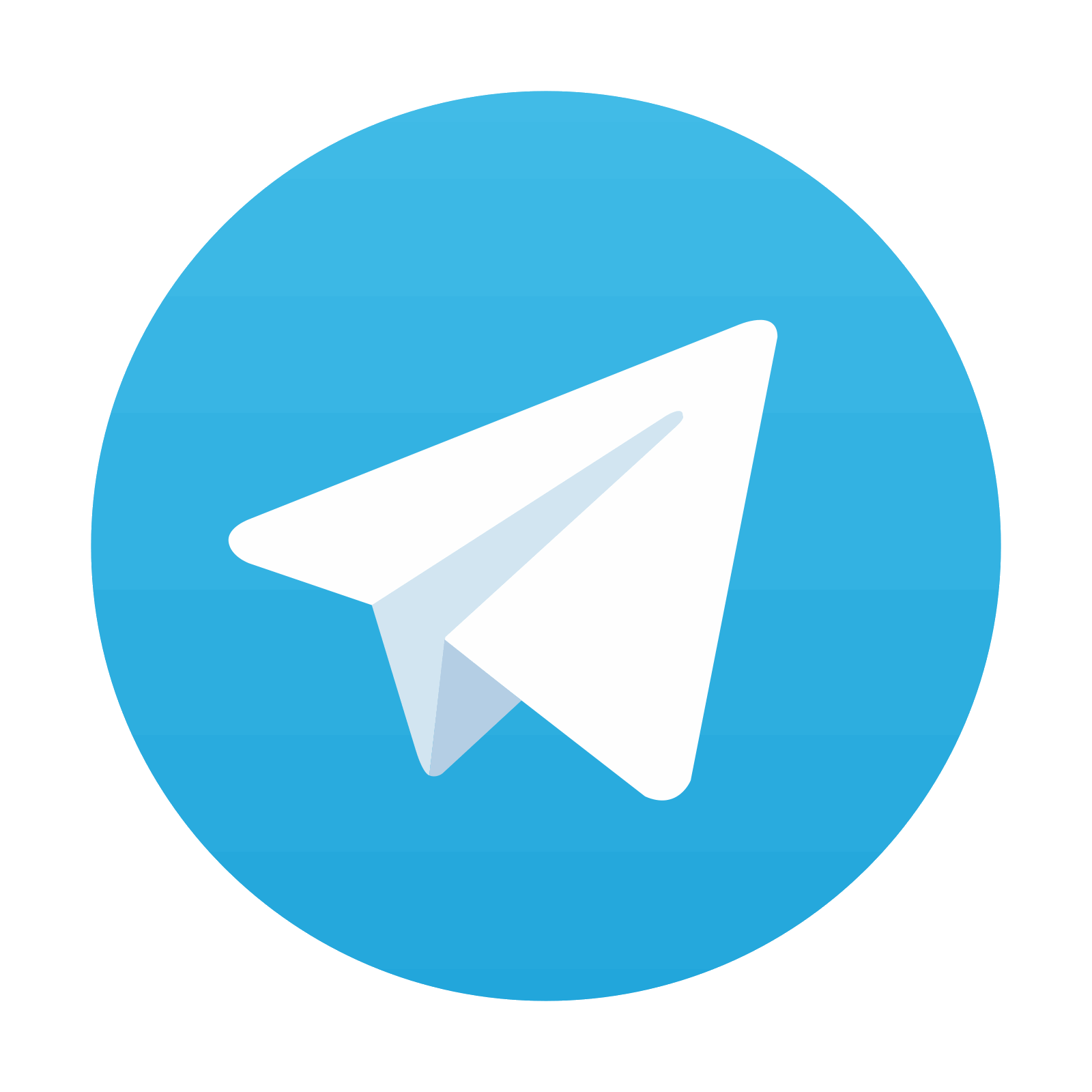
Stay updated, free articles. Join our Telegram channel
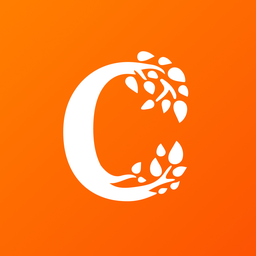
Full access? Get Clinical Tree
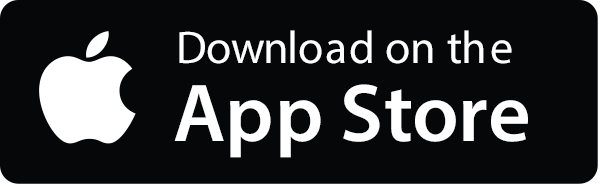
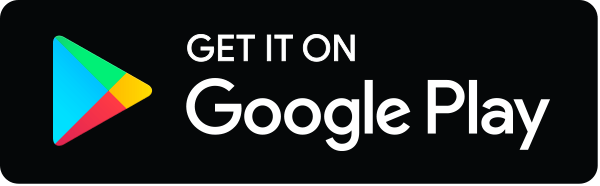