2 Physiology A. Cell membraneâa semipermeable lipid bilayer containing channel and carrier proteins that regulate the flow of ions and other molecules across the membrane. It also functions as a capacitor to store charge. B. Simple diffusionâcharacterized by kinetic movement of ions or molecules across the cell membrane without the necessity for binding to carrier proteins in the membrane. It may occur either directly through the lipid bilayer of the membrane or through protein channels that are highly selective for specific ions/molecules based on their shape, size, and charge. Simple diffusion is limited to small ions/molecules (e.g., H2O) and lipid-soluble molecules (e.g., O2, N2, CO2, and alcohols). Other molecules exhibit limited diffusion owing to their larger size (e.g., glucose) or electrostatic charge. C. Selective permeabilityâthe highly selective property of protein channels to transport specific ions/molecules across the membrane based on shape, size, and charge. For example, Na+ channels are small in diameter and contain a negatively charged inner surface to attract positively charged Na+ ions. Once a Na+ ion is inside the channel, it may then diffuse out in either direction. In contrast, K+ channels are not negatively charged and are even smaller than Na+ channels. These properties confer selective permeability to the channels for the transport of their specific ionic species. D. Gatingâmechanism responsible for controlling the permeability of a protein channel to the passage of ionic or molecular species. Gates are structural components of the protein molecule that may open or close over the opening of a channel in response to conformational changes in the protein molecule. The opening or closing of these gates is dictated by either voltage changes or the binding of ligands. 1. Voltage-gated channelsâThe ion passage of voltage-gated channels (e.g., Na+, K+, Ca2+) is regulated via conformational changes of the gate arising in response to the electrical potential across the membrane (Vm). Not all channels that pass Na+, K+, or Ca2+ are voltage-gated but for the subtypes that are, they tend to remain closed if there is a sufficiently negative charge inside the cell, and open when Vm becomes less negative (i.e., depolarized). 2. Ligand-gated channelsâLigand-gated channels open following the binding of a specific molecule (i.e., ligand) to the receptor protein. For example, acetylcholine (ACh) binds the ACh receptor, causing the gate of this channel to open; in fact, this is an important mechanism underlying the transmission of action potentials (AP) within the synapse and neuromuscular junction (NMJ). E. Facilitated diffusionâused to transport glucose and most amino acids. It involves carrier-mediated transport such that a molecule binds to a receptor protein, inducing a conformational change that carries the molecule into the cell where it is released. Upon release, the receptor protein then reverts to its original shape. The rate of diffusion is dependent upon the rate of conformational change and the number of receptors and is maximal when all carrier proteins are filled. G. Active transportâtransport of ions/molecules (e.g., Na+, K+, Ca2+, H+, Clâ, Iâ, uric acid, sugars, and many amino acids) across the cell membrane, against an electrical, chemical, or pressure gradient. This process is energy-dependent (e.g., adenosine triphosphate [ATP]). 1. Primary active transportâuses ATP-derived energy for the transport of Na+, K+, Ca2+, H+, and Clâ. The Na+/K+ pump is a carrier protein comprising two protein subunits; the larger subunit contains three receptor sites on the intracellular side for Na+, and the smaller one contains two receptor sites on the extracellular side for K+. The intracellular portion exhibits ATPase activity, such that one ATP molecule is cleaved to drive three Na+ ions out and two K+ ions into the cell. 2. Secondary active transportâuses the gradient of one compound (created by previous ATP cleavage) to drive the transport of another compound either down its electrochemical gradient (i.e., cotransport) or against it (i.e., countertransport). This process is mediated by a carrier protein. a. For example: (1) CotransportâNa+ cotransport of glucose or amino acids (2) CountertransportâNa+ countertransport of Ca2+ or H+ A. Membrane potential (Vm)âestablished by selective permeability to Na+ and K+ ions B. Nernst equationâused to determine the electric potential of a cell membrane with respect to one type of ion. Nernst potential = Âą 61 log Ci/Co, whereby Ci is the intracellular ionic concentration and Co is the extracellular ionic concentration. Note that the sign of the potential is positive when the ion is negative (e.g., Clâ), and negative when the ion is positive (e.g., Na+, K+, Ca2+). The following table illustrates ionic concentrations and equilibrium potentials. C. Equilibrium potentialâVm at which no net diffusion of the ion occurs because of balanced electrical and chemical gradients, such that the net current of the ion across the membrane is 0. D. Goldman equationâused to determine the potential across a cell’s membrane taking into account all of the ions that are permeable through that membrane: Vm = â61 log ([Na+]I Ă PNa+ + [K+]I Ă PK+ + [Clâ]o Ă PClâ)/([Na+]o Ă PNa+ + [K+]o Ă PK+ + [Clâ]I Ă PClâ). Vm is dependent on electrical charge, concentration, and permeability (P). E. Resting membrane potential (RMP)âis equal to â90 mV in large myelinated peripheral nerves and in skeletal muscle. The RMP is determined largely by the equilibrium potential of K+ (â94 mV) because K+ is 100 times more permeable than Na+ (which has an equilibrium potential of +61 mV). Ca2+ ions are not very permeable and have little effect on RMP. RMP is approximately â65 mV in the soma of the neuron and â55 mV in small nerve fibers and smooth muscle. F. Action potentialâAll-or-none, propagated phenomenon characterized by rapid changes in Vm, which alter permeability of Na+ and K+ voltage-gated channels. 1. Resting stateâThe RMP is â90 mV, primarily maintained by leaky K+ channels. a. Na+ and K+ permeability is dependent on changes in Vm during the AP, although Clâ permeability does not change much. At rest, K+ conductance is higher than Na+ conductance because the membrane leaks K+ ions to a greater extent than it does Na+ ions. 2. Depolarization stateâWhen a stimulus increases Vm sufficiently (i.e., by 15 to 30 mV, attaining a threshold level of approximately â65 mV), the membrane suddenly becomes permeable to Na+, resulting in further depolarization to +35 mV in large fibers and 0 mV in smaller fibers. This initiates the AP, which subsequently propagates along the neuronal axon. a. Approximately 40 to 80 excitatory postsynaptic potentials (EPSPs) are required to induce an AP. Inhibitory postsynaptic potentials (IPSPs) open K+ or Clâ channels, whereas EPSPs open Na+ channels. b. Only a small number of ions present in the cell need to cross the membrane (1/100,000,000) to depolarize it from â90 to +35 mV. c. Different neurons exhibit varying thresholds for excitation, baseline RMP, and different fre quency of discharges. d. Membrane accommodation to a stimulus occurs by a slow rise in the membrane potential that allows some Na+ gates to deactivate while others open. Thus, either a larger amplitude or a faster rise of depolarization is required to trigger an AP. e. The AP starts at the neuronal axon hillock, which contains sevenfold more voltage-gated Na+ channels; hence, it is more easily depolarized than the neuronal soma (+30 mV increase is required at the soma, compared with +20 mV at the hillock, to depolarize the membrane potential to â45 mV). f. The AP propagates as adjacent neuronal segments undergo local changes in membrane potential. Ion channels open and the impulse moves ahead in both directions. The velocity of the AP increases with increased transmembrane resistance, decreased internal resistance, and decreased membrane capacitance. Myelin further increases transmembrane resistance, decreases membrane capacitance, and is responsible for saltatory conduction of the AP. g. During the AP, many negatively charged molecules remain intracellular (i.e., proteins, phosphates, and sulfates). 3. Repolarization stateâFollowing depolarization, the membrane repolarizes in 1/10,000th of a second as Na+ channels close and K+ channels open. a. âPositive after-potentialâ represents the overshoot hyperpolarization to â100 mV, secondary to K+ channels remaining transiently open following repolarization. G. Voltage-gated Na+ channelsâcontain an outer activation gate and an inner inactivation gate. At RMP, the inactivation gate is open and the activation gate is closed. The activation gate opens when Vm attains â70 to â50 mV, allowing a Na+ influx. Both gates remain open for a few 10,000ths of a second (allowing for transient depolarization to +35 mV), after which the inactivation gate closes. The inactivation gate does not open again until the RMP is restored and the activation gate is closed. H. Voltage-gated K+ channelsâopen slowly as the membrane depolarizes such that K+ efflux occurs as the Na+ inactivation gate closes, thereby leading to repolarization. I. Tetrodotoxinâvoltage-gated Na+ channel blocker J. Tetraethylammonium (TEA)âvoltage-gated K+ channel blocker K. Clâ ionsâpassively leak into the cell (i.e., they are not actively pumped). Little change in Clâ conductance occurs during the AP. The intracellular concentration of Clâ is low because the â90 mV RMP repels them (i.e., negative charge). The Nernst potential for Clâ is â86 mV. L. Ca2+ pump and voltage-gated Ca2+ channelsâa Ca2+ pump actively transports Ca2+ out of the cell or into the endoplasmic reticulum, creating a Ca2+ gradient. Voltage-gated Ca2+ channels, also permeable to Na+, are also referred to as Ca2+ âNa+ channels. These are slow channels, unlike the fast voltage-gated Na+ channels. When less Ca2+ is in the interstitial fluid, Na+ channels open sooner (as low as â80 mV); thus, the membrane is more excitable and may reach tetany. Alternatively, increased levels of Ca2+ result in Ca2+ binding to Na+ channel proteins, which require larger depolarizing voltages to open. M. Patch clamp techniqueâused to record ion current flow (through either single or multiple channels) or potentials across membranes. Involves the approximation of a micropipette (with diameter as low as 1â2 mm) onto a cell surface, forming a membrane patch either on or off the cell. One can âclampâ the system to set a constant voltage or current. N. Heart muscleâAction potentials have a longer depolarization and plateau phase owing to the activity of fast voltage-gated Na+ channels, slow voltage-gated Ca2+ channels, and K+ channels that do not open until the end of the plateau. O. Rhythmicityârepetitive, self-induced discharges of cardiac muscle, smooth muscle, and some neurons. It is characterized by a depolarized resting state (RMP of â60 to â70 mV) and a decreased threshold for AP initiation secondary to membrane permeability to Na+ or Ca2+. 1. Normally, the delay in subsequent depolarization is due to an overshoot of K+ efflux causing hyperpolarization. As K+ conductance decreases, the membrane depolarizes, and then K+ conductance increases. The AP occurs when K+ conductance is at its lowest. P. Sphingomyelin (i.e., myelin)âinsulating layer of phospholipid that surrounds the axons of many neurons and contributes to faster neuronal conduction. Peripheral nerves have twice as many unmyelinated fibers than myelinated fibers. Nodes of Ranvier are 3 Îźm-long unmyelinated segments occurring every 1â3 mm along the axon. Saltatory conduction occurs between these nodes in myelinated fibers, making them faster and more energy efficient. Moreover, myelinated fibers exhibit faster repolarization than unmyelinated fibers; this repolarization is so fast that K+ channels minimally contribute to itârather it occurs mainly via the closing of Na+ channels. Q. Conduction velocityâIn small, unmyelinated nerve fibers, it is ~0.5 m/s; in large, myelinated nerve fibers, velocity is as high as 120 m/s. R. Excitation of a cellâOccurs following either mechanical stimulation (e.g., secondary to pressure or stretch of the skin), a chemical interaction (e.g., in interneurons and certain sensory receptors), or an electrical stimulation (e.g., in excitable cells such as neuronal, cardiac, or smooth muscle cells). S. Refractory periodâoccurs following depolarization, when Na+ channels (and certain Ca2+ channels) remain inactivated such that no amount of stimulus will trigger an AP to fire. 1. Absolute refractory periodâDuring this period, the neuron will not fire. It lasts 1/2500th of a second in large, myelinated fibers. T. Membrane stabilizersâagents that decrease excitability. They include increased serum Ca2+, decreased serum K+ (as in familial periodic paralysis), certain local anesthetics (e.g., procaine), acidosis (pH < 7.0), and hypoxia. U. Membrane destabilizersâagents that increase excitability. They include decreased serum Ca2+, increased serum K+, alkalosis (pH > 7.8, thus explaining why seizures are induced by hyperventilation), caffeine, and strychnine. A. Synapseâjunction between neurons, or between a neuron and muscle fiber, where impulse conduction occurs. A synapse functions to either transmit an impulse, block it, change it from a single event to repetitive impulses, or integrate it with other impulses. 1. Chemical synapseâmost common type of synapse in the central nervous system (CNS), exhibiting unidirectional flow. Approximately 50 chemical neurotransmitters have been found to date. 2. Electrical synapseâtubular protein channel (i.e., gap junction) between cells allowing the direct spread of ionic current from one cell to another. Typically found in cardiac and smooth muscle (but rarely in the mammalian CNS), they exhibit bidirectional flow. 3. 80â95% of axons synapse onto dendrites; 5â20% synapse onto the neuronal soma. B. Synaptic cleftâ20â30 nm space separating the presynaptic and postsynaptic terminals C. Presynaptic terminalâcontains synaptic vesicles and mitochondria to generate ATP for neurotransmitter synthesis. The presynaptic membrane also contains many voltage-gated Ca2+ channels that open with depolarization, allowing an influx of Ca2+ ions that induce neurotransmitter release by binding to release sites inside the terminal membrane. This stimulates neurotransmitter-containing vesicles to bind and fuse with the presynaptic membrane, leading to the exocytosis of a few vesicles per AP. 1. Synaptic vesicleâEach vesicle contains 10,000 molecules of ACh, or 1 quantum. The release of 1 quantum produces a miniature end-plate potential (MEPP). 2. Following the AP, Ca2+ ions are removed from the presynaptic terminal via active transport, bound to cytosol proteins and transported to storage vesicles. Upon fusing with the membrane, these vesicles reform intracellularly. 3. Presynaptic inhibitionâinhibitory effect following the discharge of inhibitory synapses (mediated by the neurotransmitter gamma-aminobutyric acid [GABA]) present on presynaptic terminal nerve fibrils just proximal to their termination on postsynaptic neurons. Anion channels, namely Clâ, open and allow Clâ into the terminal fibrils to inhibit subsequent excitation. This mechanism is typically seen in sensory pathways of the CNS. D. Postsynaptic terminalâcontains receptors with a binding component (for the neurotransmitter) and an ionophore component. The latter comprises either a chemically activated ion channel (Na+, K+, or Clâ) or a second-messenger system that activates an internal reaction within the postsynaptic neuron. E. Synaptic activityâdependent on the overall effect of excitatory and inhibitory input from presynaptic neurons onto the postsynaptic neuron 1. Excitationâfollows opening of Na+ channels, closing of K+ or Clâ channels, increasing excitatory or decreasing inhibitory receptor numbers, or modifying intracellular metabolic activity 2. Inhibitionâfollows opening of K+ or Clâ channels or modifying excitatory or inhibitory receptor numbers 3. Summationâthe additive effect of EPSPs and IPSPs, whose net effect is either to increase or decrease ion permeability in the membrane. This phenomenon may last for 1â2 ms and typically resolves within 15 ms. Each axon terminal releases substances that induce a 0.5â1 mV change in Vm; 20 mV is needed to attain the depolarization threshold required to trigger an AP. Summation may occur either in the spatial or the temporal domain. a. Spatial summation arises when presynaptic terminals residing in different parts of the neuron are stimulated simultaneously. b. Temporal summation is the additive effect of postsynaptic potentials over time. A neuron is considered âfacilitatedâ if an EPSP puts it closer to threshold. 4. Decremented conductionâMany dendrites are unable to generate APs because they have too few voltage-gated channels, yet electrotonic current may spread to the axon by means of the cytosol. Decremented conduction occurs because changes in Vm more distal on the dendrite have less effect on the soma due to current leaking out along the way. 5. Fatigueâwhen repetitive stimulation leads to a decreased frequency of discharge. This is a protective mechanism against excessive neuronal activity. It arises following exhaustion of neurotransmitter stores in presynaptic terminals (because neurons only store sufficient transmitter for ~10,000 synaptic transmissions), progressive inactivation of postsynaptic receptors and by accumulation of postsynaptic Ca2+, which opens Ca2+-activated K+ channels that hyperpolarize the membrane. 6. Posttetanic facilitationâoccurs in the context of repetitive impulses inducing an excitatory response, followed by a transient period of rest during which the synapse remains more responsive to subsequent stimulation than is normally the case. This occurs because repetitive stimuli lead to Ca2+ build-up in the presynaptic terminals, ultimately causing increased neurotransmitter release. 7. Synaptic delayâthe time for synaptic transmission (of an AP) to occur from presynaptic to postsynaptic terminal; typically 0.5 ms duration 8. Approximately 50 synaptic transmitters have been identified; these are classified as (1) small-molecule, rapidly acting neurotransmitters, and (2) neuropeptides. F. Small-molecule neurotransmittersârapidly acting substances that account for most of the acute reactions in the CNS. They are produced in the cytosol of presynaptic terminals and are actively transported into vesicles, a few of which are released per AP. These agents usually act upon ion channels, a process that occurs within milliseconds. They may also activate receptor-linked enzymes and are ultimately recycled via active transport into presynaptic terminals (i.e., reuptake) for subsequent use. Only a single type of small-molecule neurotransmitter is released per neuron, yet the same neuron may also release more than one neuropeptide. a. Acetylcholineâusually excitatory. ACh is produced in the presynaptic terminal from acetyl-co-enzyme A (CoA) and choline, and is stored in vesicles. Upon release, it is split in the synaptic cleft by acetylcholinesterase. ACh is found in the motor cortex, skeletal muscle, preganglionic autonomic nerves, postganglionic parasympathetic nerves, and postganglionic sympathetic nerves supplying sweat glands. b. Norepinephrineâusually excitatory; found in the pontine locus ceruleus and postganglionic sympathetic nerve fibers c. Dopamineâinhibitory; found in the neurons of the substantia nigra (SN) that project to the putamen and caudate. Synthesis occurs following conversion of tyrosine to 3,4-dihydroxy-L-phenylalanine (DOPA) by the enzyme tyrosine hydroxylase (i.e., the rate-limiting step), followed by conversion to DA. This is subsequently converted to NE, and finally to EPI. d. Glycineâinhibitory; found in the spinal cord (Renshaw cells) e. GABAâinhibitory; found in the cortex, basal ganglia, cerebellum (Purkinje cells), and spinal cord f. Glutamateâexcitatory; found in the cortex, dentate gyrus of the hippocampus, striatum, and cerebellar granule cells g. Serotoninâinhibitory; found in brainstem nuclei (median raphe nuclei) that project to the hypothalamus and spinal cord (dorsal horns). It acts to inhibit pain pathways, induces sleep, and affects mood. It is used by the pineal gland to synthesize melatonin, which is released in a diurnal fashion. 2. Small-molecule neurotransmitter receptors a. ACh receptors (1) Nicotinic receptorsâStimulated by nicotine, they are located in the NMJ and preganglionic endings of both sympathetic and parasympathetic fibers. Autonomic nicotinic receptors are composed of five subunits (i.e., 2 alpha, [Îą2], one beta [β], one gamma [Îł], and one delta [δ]). The Îą-subunit is composed of four hydrophobic transmembrane proteins and serves as the binding site for ACh; hence, each receptor binds two ACh molecules. Stimulation of the receptor generates a fast EPSP. The receptor is blocked by hexamethonium in a depolarizing fashion that cannot be reversed by anticholinesterase. The nicotinic receptor at the NMJ consists of only two Îą-subunits, and stimulation of the receptor elicits a slow EPSP (by opening both Na+ and Ca2+ channels) and a slow IPSP (by opening K+ channels). (2) Muscarinic receptorsâStimulated by muscarine, they are located in postganglionic para-sympathetic endings and postganglionic sympathetic endings innervating sweat glands. The receptorsâ effects are mediated by a G protein via a second messenger system, and activation of one G protein inhibits all other G proteins in the cell. These receptors are blocked by pertussis toxin. b. Adrenergic receptorsâNE stimulates Îą-receptors to a greater extent than β-receptors. EPI stimulates Îą- and β-receptors to the same extent. See Chapter 6 (Critical Care) for more details. c. DA receptorâDA1 receptor functions via cAMP (i.e., second messenger). d. Glycine receptorâinhibitory; involves Clâ channels and is bound by strychnine (i.e., competitive antagonist). Mutations cause increased muscle rigidity (as in stiff person syndrome). f. Glutamate receptorsâLinked to cell death (via Ca2+ influx) and synaptic plasticity, these receptors are either ligand-gated (e.g., metabotropic) or a combination of ligand- and voltage-gated [e.g., N-methyl-D-aspartic acid (NMDA), Îą-amino-3-hydroxy-5-methylisoxazole-4-propionic acid (AMPA)/quisqualate, and kainate]. Some are blocked by Mg2+. The NMDA receptor is one type of glutamate receptor. It is voltage-regulated because at RMP, Mg2+ blocks the channel, whereas with depolarization, Mg2+ is driven out and the channel opens. It is also ligand-gated by gluta-mate and permeable to Ca2+, Na+, and K+. It requires glycine as a coagonist for activation. NonNMDA glutamate receptors do not require glycine for activation. The AMPA/quisqualate and kainate receptors are permeable to monovalent cations (e.g., Na+ and K+). G. Neuropeptide neurotransmittersâhigher molecular weight and slow-acting. These transmitters are produced in the soma and packaged by the endoplasmic reticulum and Golgi apparatus after being cleaved from larger peptides. Vesicles of these transmitters are then transported to presynaptic membranes via axonal streaming, at a rate of a few cm/day. The vesicles are not recycled, and although fewer are released in comparison to small-molecule transmitters, they are longer-acting and significantly more potent. Neuropeptides close Ca2+ channels and alter metabolic machinery and receptors. They can diffuse into tissues and are typically destroyed within minutes to hours. Examples include hypothalamic-releasing hormones, pituitary peptides, cholecystokinin (CCK), and bradykinin. H. Intracellular second messengers 1. cAMPâincreased with DA1 receptor stimulation 2. Cyclic guanosine monophosphate (cGMP)âinvolved with photoreception; increased by NO 3. Inositol triphosphate (IP3)âhydrolyzed by phospholipase C; opens Ca2+ release channels in the endoplasmic/sarcoplasmic reticulum, thereby increasing Ca2+ in the cytosol 4. Diacylglycerol (DAG)âsynergistically activates protein kinase C with Ca2+ 5. Ca2+âbinds calmodulin 6. G proteinsâsee Section VII I. Axonal transportâmay be slow (several mm/day) or fast (200â400 mm/day, via microtubular mechanisms) and either anterograde or retrograde. The proteins kinesin and dynein mediate anterograde and retrograde axonal transport, respectively. A. Sensory receptors (Fig. 2.1) 1. Mechanoreceptorsâsense mechanical deformation Fig. 2.1 Receptors of the somatosensory system. (A,B) Skin receptors include Meissner, Ruffini, and Vater-Pacini corpuscles as well as bare nerve endings for nociception and temperature and several other receptors. (C) Proprioception encompasses position, force, and motion sense. Proprioceptors include muscle spindles, tendon sensors, and joint sensors (not shown). (From THIEME Atlas of Anatomy, Head and Neuroanatomy, Š Thieme 2007, Illustration by Markus Voll.) 2. Thermoreceptorsâsense changes in temperature; may be specific for heat or cold 3. Nociceptorsâgenerate sensation of pain in the context of tissue damage or physical/chemical changes 4. Electromagnetic receptorsâdetect light in the retina 5. Chemoreceptorsâdetect various chemicals pertaining to taste, smell, arterial O2 and CO2, blood osmolarity, etc. C. Receptor potentialâWhen the receptor potential rises above threshold, an AP is generated. A higher receptor potential elicits increased impulse frequency but does not change impulse amplitude. The receptor potential is graded and nonpropagated, in contrast to the AP, which is a propagated, all-or-none response. D. Receptor potential changes via different mechanisms 1. Mechanoreceptorsâreact as deformation stretches the membrane and opens ion channels a. For example: (1) The Pacinian corpuscle consists of a central nerve fiber surrounded by concentric capsular layers. Mechanical compression (i.e., elongation, bending, denting, etc.) induces changes in the central fiber. As the fiber’s unmyelinated tip deforms, Na+ conductance increases, a receptor potential is generated, and an AP ensues if the receptor potential is sufficiently large to reach threshold. The frequency of the AP is initially proportional to the amplitude of the receptor potential but plateaus at high levels. 2. Chemical receptorsâstimulated by certain chemical agents, thereby triggering specific ion channels to open 3. Temperature receptorsâreact to temperature changes by directly opening ion channels or indirectly altering membrane ion permeability 4. Electromagnetic energyâdirectly opens ion channels or indirectly alters membrane ion permeability E. Adaptationâoccurs when a sensory receptor decreases its firing rate in the context of continuous sensory stimulation 1. For example, a Pacinian corpuscle adapts as follows: a. Following the initial pressure wave, fluid within the corpuscle is evenly distributed such that firing ceases even though the corpuscle is still compressed; another signal develops when the compression is removed. Such adaptation occurs in 1/100 second. b. Accommodation via the gradual inactivation of Na+ channelsâThis adaptation is much slower. Both the receptor and the terminal nerve fibers can adapt via this mechanism. 2. Mechanoreceptors may adapt completely; pain receptors tend to adapt to a lesser extent and chemoreceptors vary in their ability to adapt. 3. Rapidly adapting (phasic) versus slowly adapting (tonic) receptors a. Pacinian corpuscles and hair receptors are phasic receptors; they react only to changes in stimulus strength and are incapable of transmitting continuous signals. Moreover, they increase their firing rate in response to increased rates of change in stimulation and can rapidly adapt. b. Joint capsules, muscle spindles, vestibular maculae, pain receptors, baroreceptors, chemoreceptors, Ruffini end organs, and Merkel disks are tonic receptors. They adapt slowly, transmit impulses for many hours, and rarely adapt to extinction. A. Neuronal axon fibers are 0.2â20 mm in diameter. The velocity of transmission ranges from 0.5â120 m/s and is fastest in large fibers. Of interest, large fibers conduct impulses over a distance equivalent to the length of a football field per second, while small fibers require up to 2 seconds to transmit impulses from the big toe to the spinal cord. B. Types of sensory nerve fibers 1. Ia (Îą-type A)âannulospiral endings of muscle spindles; largest (i.e., 17 Îźm diameter) and fastest (120 m/s) 2. Ib (Îą-type A)âGolgi tendon organs; 16 Îźm in diameter 3. II (β- and Îł-type A)âflower-spray endings of muscle spindles and cutaneous tactile receptors; 8 Îźm in diameter 4. III (δ-type A)âtemperature, crude touch, and pricking pain; 3 Îźm in diameter 5. IV (type C)âunmyelinated fibers relaying pain, itch, temperature, and crude touch sensations; 0.5â2 Îźm in diameter C. Types of motor nerve fibers 1. Skeletal muscleâÎą-type A (myelinated; fastest fibers) 2. Muscle spindleâÎł-type A (myelinated) 3. Sympatheticâtype C (unmyelinated; slowest fibers) D. Neuronal circuits and information processing 1. Receptive field of a nerve fiberâthe surface area it innervates. Each nerve fiber has multiple endings and most are in the center of the receptive field. 2. Spatial summation of a stimulusâThe more intense a given stimulus, the greater the number of sensory nerve fibers activated. Thus, increased signal strength is relayed via progressively larger numbers of activated fibers. 3. Temporal summationâoccurs when increased stimulus intensity increases the rate of neuronal firing 4. Neuronal poolârefers to a specifically organized, collective group of input and output neuronal fibers that processes signals in its own distinct way. Input fibers may have sufficiently extensive branches to relay suprathreshold stimuli into the center of the stimulatory field supplied by the neuronal pool, yet relay only subthreshold (i.e., facilitated) stimuli to the periphery. The center of the stimulatory field is the âliminalâ or âstimulated zoneâ and the peripheral area is the âsubthresholdâ or âsubliminal zone.â 5. Divergenceâoccurs when an input fiber activates greater numbers of nerve fibers leaving the neuronal pool. In other words, one neuron synapses onto multiple cells, either amplifying a signal in one tract (e.g., one motor neuron stimulating 10,000 muscle fibers) or multiple tracts (e.g., dorsal column input into the cortex and cerebellum). 6. Convergenceâoccurs when multiple different neuronal fibers, either from similar or different sources, synapse onto one neuron. Rarely, a single presynaptic neuron is able to sufficiently stimulate a postsynaptic neuron to elicit an AP (e.g., Purkinje cells synapsing onto deep nuclei in the cerebellum). 7. Reciprocal inhibitory circuitâan axonal branch that stimulates an interneuron in the pathway, which subsequently inhibits an antagonist muscle group while simultaneously stimulating an agonist muscle group 8. After dischargeâarises in the context of long-acting neurotransmitters, when lingering stimulatory input continues to elicit APs despite removal of the initial signal 9. Oscillatory (reverberatory) circuitâa circuit whose neuronal output feeds back to reactivate the circuit. A longer period between initial activation and the feedback cycle develops if more interneurons are integrated into the circuit. Synaptic fatigue ultimately stops the reverberatory cycle. 10. Signal output occurs either continuously or rhythmically. a. Continuous signal output arises with increased neuronal excitability caused by low RMPs (e.g., cerebellar neurons and spinal cord interneurons) and in reverberating circuits where input either increases or decreases the frequency of AP firing (e.g., autonomic nervous system). b. Rhythmic signal output occurs in reverberating circuits (e.g., respiratory center of the pons and medulla). 11. Neuronal circuit stabilityâNeuronal activity is organized and controlled by a combination of excitatory and inhibitory circuits; otherwise, signal transmission would be disrupted and hyperexcitability (i.e., seizures) could develop. Inhibitory mechanisms include a. Inhibitory (i.e., negative feedback) circuits that inhibit input neurons or interneurons b. Synaptic fatigue arising from ionic changes (in the short term) and upgrading/downgrading of receptor proteins (in the long term) A. Tactile sensesâtouch, pressure, vibration, and tickle senses. Touch, pressure, and vibration use the same receptor pathways. B. Position sensesâstatic position and rate of movement senses C. Somatic sensory receptors 1. Free nerve endingsârespond to pain, touch, and pressure 2. Meissner corpusclesârespond to touch. These are rapidly-adapting receptors located superficially in dermal papillae of non-hairy skin (e.g., fingertips, lips). They have small receptor fields and transmit signals via large, myelinated β-type A fibers. 3. Merkel disks (i.e., expanded tip tactile receptors)ârespond to touch and pressure. These are slowly-adapting receptors with small receptive fields, located superficially in the dermal papillae of hairy and non-hairy skin. Merkel disks typically group together to fill a single receptor organ underneath the epithelium, the Iggo dome receptor, which is innervated by a single, myelinated, β-type A fiber. 4. Pacinian corpusclesârespond to vibration (i.e., high-frequency stimulation). These are rapidly-adapting receptors and are located in superficial and deep tissues. 5. Ruffini end organsârespond to heavy touch and pressure. These are slowly-adapting receptors located in deep layers (i.e., subcutaneous tissue and joint capsules) and exhibit large receptive fields. 6. Hair end organsârespond to touch. These are rapidly-adapting receptors located at the base of hair follicles. D. Somatic nerve fibers 1. Touchâmostly relayed by β-type A fibers at speeds of 30â70 m/s. Free nerve endings also transmit touch sensations via myelinated δ-type A fibers at speeds of 5â30 m/s and tickle sensations via unmyelinated C fibers at speeds of 62 m/s. Crude pressure, poorly localized touch, and tickle sensations are relayed via smaller, slower fibers occupying less space in the nerve bundle. 2. Vibrationârelayed by β-type A fibers. All tactile receptors contribute, each at different frequencies (e.g., Pacinian corpuscles at 30â800 cycles/s, Meissner corpuscles at 80 cycles/s). 4. Painâsensed by free nerve endings and is transmitted via C fibers. See the following sections for more detail. 5. Peripheral sensory or mixed nervesâcontain fourfold more unmyelinated fibers than myelinated fibers. The unmyelinated fibers are C fibers; the myelinated fibers are δ-type A fibers. Proprioceptive fibers travel with motor nerves. Each axon connects to several receptors of one type. E. Anterolateral ascending sensory systemâcontains smaller myelinated fibers that conduct at speeds of 2â40 m/s. It relays warm, cold, pain, crude touch, tickle, itch, and sexual sensations. These sensations do not require discrete localization, and thus they have poor spatial localization and intensity grading and slow signal repetition. Crude touch originates from laminae 1, 4, 5, and 6 in the dorsal horns. Fibers ascend in the anterior (i.e., ipsilateral) and lateral (i.e., contralateral, due to crossing of fibers in the anterior commissure, within three levels) spinothalamic tracts to the ventroposterolateral (VPL; body), ventroposteromedial (VPM; face), and posterior thalamic nuclei for touch and temperature sensations. Fibers also relay impulses in the spinoreticular tract to the intralaminar thalamic nucleus for pain. F. Dorsal column systemâcontains large myelinated fibers that conduct at speeds of 30â110 m/s. It relays fine touch, vibration, position, and pressure sensations. Moreover, its fibers exhibit a greater extent of spatial orientation (i.e., somatotopic organization). 1. Axons enter the spinal cord and divide into a medial branch that travels up the dorsal column (25% of fibers) and a lateral branch (75% of fibers) with multiple synapses in the dorsal horn for reflexes. Second-order neurons are located in the medulla, within the medially situated nuclei gracilis (responsible for the lower limbs) and laterally situated nuclei cuneatus (responsible for the upper limbs). Arcuate fibers cross to form the medial lemniscus (ML), joining fibers from the main sensory nucleus of the trigeminal nerve (V) and the upper spinal nucleus of V to terminate in the thalamic VPL (body) and VPM (face). 2. The ventrobasal complex (VPL, VPM, and the posterior thalamic nucleus) sends fibers to the cortical somatosensory areas S1 and S2. A somatotopic organization exists with the lower limbs represented medially in the spinal cord, laterally in the thalamus, and medially again in the cortex (Fig. 2.2). 3. Position senseâstatic and kinesthetic proprioception to detect joint angles in all directions and the positional rate of change, respectively 4. Proprioceptive impulsesâbegin at the muscle spindles, Pacinian corpuscles, Ruffini end organs, and Golgi tendon organs of the extremities. Lower limb proprioception is conveyed within the lateral column from Clarke column neurons through the dorsal spinocerebellar tract to the cerebellum (i.e., not in the posterior columns). Upper extremity proprioception is conveyed through the posterior columns in the fasciculus cuneatus prior to synapsing onto the accessory cuneate nucleus in the caudal medulla, before being relayed to the cerebellum via the cuneocerebellar tract. G. Sensory cortexâNeuronal cells are arranged in vertical columns of 10,000 neurons, each column of diameter 0.3â0.5 mm and able to detect one sensory modality. Different modality columns are interspersed with one another. 1. Six layers of neurons in the cerebral cortex a. Layers 1 (most superficial) and 2 receive diffuse, nonspecific input from the lower brain and may control the excitability of a region. Fig. 2.2 Somatotopic organization of specific thalamic nuclei. Transverse section. Ventrolateral thalamic nuclei receive afferents from the crossed superior cerebellar peduncle and relay to the cortex. The lateral part of the ventrolateral nucleus relays impulses from the extremities and the medial part relays impulses from the head. The ventral intermediate nucleus receives vestibular input. The posterior column input is via the medial lemniscus to the ventral posterolateral nucleus, and trigeminal fibers from the head enter the ventral posteromedial nucleus. (From THIEME Atlas of Anatomy, Head and Neuroanatomy, Š Thieme 2007, Illustration by Markus Voll.) b. Layers 2 (association fibers) and 3 (association and commissural fibers) relay axons to other cortical areas. c. Layer 4 of each column does not interact with the other columns, whereas other layers do. Afferent fibers arrive in layer 4 and either spread up or down a column. d. Layers 5 and 6 (projection fibers) disperse axons to distant parts of the nervous system. Layer 5 is larger and connects to the brainstem and spinal cord, whereas layer 6 is smaller and connects to the thalamus. 2. Different somatic cortical regions regulate different areas of the body; the size of these cortical regions is dependent upon sensory receptor numbers in these various body sites. Furthermore, all sensory pathways contribute to lateral inhibition of adjacent neurons, either via interneurons or presynaptic inhibition, to increase the degree of contrast between activated and inhibited neuronal pools in that region. 3. Intensity discriminationâdecreases as stimulus intensity is increased. However, percent change is more important than total stimulus intensity; thus, in the context of an increased intensity of stimulation, a greater percent change in stimulus is required for that stimulus to be detected. 4. Primary somatosensory area (S1, Brodmann areas 1, 2, 3)âlocalizes sensations and detects pressure, weight, shapes, and textures. No pain or temperature loss arises if S1 is removed; however, localization is diminished. The facial region above the nose is represented bilaterally; also, the lips have the largest area of representation. Brodmann area 1 receives input from rapidly-adapting skin receptors; area 2 from deep pressure and joint position receptors; area 3a (located anterior and deep in the central sulcus with connections to the motor cortex) from muscle, tendon, and joint stretch receptors; and area 3b from both slowly- and rapidly-adapting skin receptors. Activation of these receptors elicits contralateral sensory perception. 5. Secondary somatosensory area (S2)âlocated posterolateral to S1 on the superior bank of the sylvian fissure. It has poor primary sensory representation but is required for shape discrimination, at least in animals. The lower limbs are represented medially. Stimulation elicits bilateral sensory perception in this region. 6. Somatic association areas (Brodmann areas 5, 7)âlocated behind S1 and above S2. Receives input from S1, ventrobasal thalamus (VPL, VPM, posterior thalamic nucleus), visual cortex, and auditory cortex. Stimulation elicits complex body sensations. A lesion in this region results in amorphosynthesis (i.e., the inability to recognize or detect objects sensed contralaterally) and astereognosia. This region contributes to two-point discrimination (1 mm in the finger, 30â70 mm in the back). 7. Thalamusâdiscriminates pain and temperature (and to a lesser extent, touch). Inhibitory corticofugal fibers synapse onto peripheral relay nuclei in the thalamus, medulla, and spinal cord to regulate sensitivity to input, to decrease lateral spread (and thus increase signal contrast), and to maintain the sensory system in an appropriate operating range of sensitivity. H. Pain and temperature sensation 1. Pain serves a protective function and, unlike sensations other than vision (otherwise one would be blind in continuous light), is nonadapting. It may be classified as follows: a. Fast painâoccurs in 0.1 second, travels 6â30 m/s, and is sharp and electric in quality. It is relayed via δâtype A fibers and is detected by mechanical and thermal receptors in the skin. b. Slow painâoccurs after 1 second but increases over seconds to minutes, travels 0.5â2 m/s, and is burning, aching, and/or throbbing. It is transmitted by C fibers, detected by all receptors in the skin and deep tissues, and generally associated with tissue destruction. 2. Pain receptorsâfree nerve endings located in the skin, periosteum, arterial walls, joint surfaces, and dura. They respond to mechanical, thermal, and chemical stimuli to varying degrees and exhibit very little adaptation to these stimuli. In fact, pain receptors may even become more sensitive (i.e., hyper-algesic) to persistent stimuli. Furthermore, increased pain intensity reflects increased tissue damage. 3. Pain pathway mediatorsâbradykinin, 5-hydroxytryptamine (5-HT; serotonin), histamine, K+, acids, acetylcholine (ACh), and proteolytic enzymes all activate pain receptors. Prostaglandins enhance pain receptor sensitivity but do not themselves excite these receptors. 4. Potential causes of pain a. Thermal painâactivated at ⼠45°C, at which point tissue damage begins b. Tissue ischemiaâPain results from altered tissue metabolism, likely attributed to the production of lactate (i.e., acidosis). c. Spasm of a muscle, artery, or hollow viscusâinduces pain by stimulating mechanoreceptors or indirectly compressing blood vessels, resulting in ischemia. Spasmodic activity increases metabolism in muscle tissue, contributing to ischemia and releasing chemical mediators of pain. d. Neurogenic inflammationâelicited by antidromic APs from spinal ganglia stimulating the release of substance P from C fiber terminals in the skin. Erythema and edematous changes ensue following histamine release. 5. Relevant anatomy a. Afferent pain fibersâenter the spinal cord to ascend/descend 1â3 segments in Lissauer tract (i.e., posterior to the dorsal horn), prior to terminating in the dorsal horn itself b. Neospinothalamic tractârelays fast pain with better localization than for slow pain (but only to ~10 cm, unless tactile sensation is also activated). It is composed of δ-type A fibers receiving sensory input from mechanical and thermal receptors and relays impulses (via glutamate, a fast-acting transmitter) to second-order neurons in lamina 1 (i.e., lamina marginalis). These cross in the anterior commissure of the spinal cord, ascend in the anterolateral tracts to synapse onto third-order neurons in the ventrobasal thalamus, and then continue on to fourth-order neurons in the somatosensory cortex. c. Paleospinothalamic tractâtransmits slow pain with poor localization, due to its diffuse connectivity. It consists of C fibers synapsing onto second-order neurons in laminas 2 and 3 (i.e., substantia gelatinosa) via glutamate and substance P (i.e., a slow-acting transmitter), ultimately relaying signals to laminas 5â8. From here, impulses cross in the anterior commissure (although some remain ipsilateral) and ascend to third-order neurons in the reticular formation, tectum, and periaqueductal gray matter; only 10â25% continue to terminate in the thalamus. The reticular formation then relays fibers to the thalamic intralaminar nuclei, hypothalamus, and basal brain, accounting for the strong arousal (and suffering) associated with pain. Note that conscious pain may be perceived without cortical processing of painful stimuli, but the cortex likely helps to qualify such sensations. 6. Analgesia system a. Periaqueductal gray matter and periventricular hypothalamusâcontain neurons whose axons release enkephalins onto the nucleus raphe magnus (lower pons and upper medulla) and the nucleus reticularis paragigantocellularis (lateral medulla). Secondary neurons release 5-HT to a pain inhibition complex in the dorsolateral spinal cord. Ultimately, the analgesia system blocks pain before it is relayed to the brain. (1) Enkephalins cause presynaptic inhibition of C and δ-type A fibers in the dorsal horns by blocking Ca2+ channels. These enkephalins mediate presynaptic pain inhibition, particularly in laminas 1, 2, and 5. Neurons in laminas 1 and 5 possess presynaptic and postsynaptic opiate receptors. NE from the pons also decreases pain, thus accounting for stress-induced analgesia. (2) Endogenous opiatesâβ-endorphin (i.e., hypothalamus and pituitary), met-enkephalin and leu-enkephalin (i.e., spinal cord system), and dynorphin (200 times more potent than morphine) b. âGate controlâ theoryâintroduced by Melzack and Wall in 1965. Large myelinated fibers have negative dorsal root potentials, and smaller C fibers have positive potentials. Stimulation of the larger fibers prevents transmission of pain impulses in the smaller fibers by maintaining a negative potential in the dorsal horns. One problem with this theory is that the loss of large myelinated fibers does not result in increased pain. However, electrical stimulation of large fibers by transcutaneous electrical nerve stimulation (TENS) units, spinal cord stimulators, or deep brain stimulators does appear to cause lateral inhibition of pain. Acupuncture may also work via this mechanism, as well as by endogenous opiates and psychogenic input. c. Medical treatment for painâAmitriptyline decreases pain by stimulating 5-HT release from the descending pathway and increasing NE release. Nonsteroidal antiinflammatory drugs (NSAIDs) decrease the production of pain-stimulating prostaglandins. d. Deep brain stimulation of the periventricular and periaqueductal gray matter has been used to treat chronic pain. Side effects of periaqueductal gray matter stimulation are diplopia, oscillopsia, fear, and anxiety. Stimulation of periventricular gray matter is much better tolerated. 7. Referred painâVisceral pain fibers synapse onto the same secondary neurons in the spinal cord (lamina 5) as do fibers from the skin, such that they conduct via the same central pathway. The only visceral receptors that reach consciousness are for pain. a. Visceral painâdifferent from surface pain. Focal injuries are not very painful, but diffuse injuries (e.g., ischemic gut) certainly are, mediated by pain-relaying C fibers that travel with sympathetic (occasionally parasympathetic) nerves. b. Visceral pain is triggered by ischemia, chemical damage, spasm, distention, and ligamentous stretching. Spasm and distention cause both mechanical pain and ischemia. The liver parenchyma and lung alveoli are insensitive to pain, but the bronchi, parietal pleura, liver capsule, and bile ducts are all sensitive. d. From an embryological standpoint, the heart originates in the neck/upper thorax of the embryo; thus, visceral pain is referred to C3âT5 (i.e., neck, shoulder, and arm). Pain is also more fre quently left-sided than right-sided because left-sided vessels (e.g., aorta) are more often occluded by coronary artery disease. e. Visceral pain is localized by two transmission pathways. (1) âTrueâ visceral painâreferred pain to body surface areas, dependent on sensory C fiber transmission to autonomic nerves (2) Parietal sensationsâIrritated parietal surfaces relay pain directly into spinal nerves. For example, appendicitis typically begins with periumbilical pain from C fibers, progressing to right-sided, lower-quadrant pain from δ-type A fibers. These help to localize the pain once the inflamed appendix irritates the overlying peritoneum. 8. Pain syndromes a. Hyperalgesiaâincreased sensitivity (i.e., decreased threshold) to pain (1) Primary hyperalgesiaâincreased sensitivity of receptors (e.g., sunburn, due to histamine release from damaged tissue) (2) Secondary hyperalgesiaâdue to facilitation resulting from lesions in the spinal cord or thalamus b. Hyperpathiaâincreased reaction to pain, but with increased threshold c. Allodyniaâpain triggered by typically nonpainful stimuli (e.g., light touch) d. Reflex sympathetic dystrophyâmay develop following neuronal damage, with the sprouting of axons that are sensitive to EPI or NE e. Phantom painâmay arise in the absence of normal input, when pain-related neurons in lamina 5 (and later the thalamus) fire spontaneously f. Thalamic pain (DejerineâRoussy) syndromeâusually due to a posteroventral thalamic stroke causing ataxia and contralateral hemianesthesia, which in subsequent weeks to months is characterized by a return of crude sensation, but also increased pain and affective, unpleasant feelings. It may be caused by facilitation of the medial thalamic nucleus with increased transmission of reticular formation pain. g. Shingles (herpes zoster)âPain is triggered by irritation of the dorsal root ganglion cells, following localized infection with the herpesvirus. 9. Headacheâcaused by referred pain from sinuses, temporomandibular joint, ocular structures, dura, blood vessels, or other deep structures. The brain itself is insensitive to pain, but the sinuses, tentorium, dura, and vessels are all sensitive. a. Innervation (1) Supratentoriallyâby the trigeminal nerve; manifests as frontal headache (2) Infratentoriallyâby C2, cranial nerve (CN) IX, and CN X; manifests as occipital and retroauricular headache b. Postlumbar puncture headacheâcaused by a decreased cerebrospinal fluid (CSF) volume that allows the weight of the brain to stretch blood vessels bridging from the surface of the brain to the skull c. Migraine headachesâlikely a vascular phenomenon; caused by reflex spasm of intracranial arteries leading to ischemia, followed by dilatation for 24â48 hours, with increased blood flow and arterial wall stretching that produces a throbbing type of headache d. Postethanol or âhangoverâ painâdue to chemical irritation of the meninges f. Muscle spasmsâproduce referred pain over the scalp g. Nasal structuresâmay become inflamed, causing headache h. Eye activityâCiliary muscle contraction (i.e., attempting to obtain focused vision) and reflex vasospasm may cause a retroorbital headache. 10. Thermal sensationâdetected by three receptors: a. Cold receptorsâmore numerous; consist of myelinated δ-type A fibers terminating in the basal epidermal cells, and some free C fiber endings b. Warm receptorsâfree nerve endings from C fibers; stimulated if the temperature is >30 °C c. Pain receptors for extreme temperatures (i.e. <10 °C or >45 °C)âThese receptors are in the subcutaneous tissue and separate from each other. The receptive field is 1 mm in diameter and detects a change in temperature with slow adaptation, activating receptors by changing the metabolic rate of neurons. Fibers enter the spinal cord, travel in the Lissauer tract; synapse in laminas 1, 2, and 3 (i.e., same as pain); cross to the contralateral side; and ascend to the reticular formation, ventrobasal thalamus, and S1 cortex. A. Physics of vision 1. Speed of lightâ300,000 km/s in a vacuum; slightly less in air and much less in a liquid or solid 2. Refractionâbending of light rays at an interface between substances of different density. Refraction depends on the angle between the interface and the wave front (e.g., no refraction occurs if these are perpendicular), and the ratio of the two refractive indices. Refractive index = Velocity in air/velocity in a substance. The refractive index of air is 1. 3. Convex lensâRays converge at a focal point beyond the lens. 4. Concave lensâRays diverge, with the focal point before the lens. 5. Focal length (f)âdetermined via the equation: 1/f = 1/a + 1/b, where a = distance from a point source to the lens and b = distance of focus 6. Refractive powerâincreases as the lens bends rays to a greater extent. It is measured in diopters (D) = 1/f (in meters). +1 D means the focal point is 1 m beyond a convex lens, +2 is 0.5 m, and +10 is 0.1 m. With a concave lens, the diopter number is negative. 7. The eye functions as a camera with four refractive indices: air/cornea, cornea/aqueous humor, aqueous humor/lens, and lens/vitreous humor. Most refraction occurs at the air/cornea interface because the other components are similar in density. The lens is less refractive as it is surrounded by fluid, but it is necessary for accommodation. The image on the retina is inverted and reversed. The eye unit has a maximum refraction of 59 D. Refractive power in children ranges from 20 to 34 D, with an accommodation of 14 D. 8. Depth perception is achieved by: a. Subconsciously comparing a visualized object’s size against the expected size b. Moving parallaxâNearby objects move more in the visual field when the head is turned. c. Stereopsisâbinocular vision, whereby an image strikes both eyes at different retinal sites B. Anatomy of the eye 1. Lensâa strong, elastic capsule filled with proteinaceous transparent fibers. When it is relaxed, it is spherical in shape. Seventy ligaments (zonules) are radially attached to the lens and the ciliary body at the anterior border of the choroid, to provide constant tension to maintain the lens in a flat shape. 2. Ciliary bodyâhas ciliary muscles with two sets of fibers: meridional fibers that attach to the corneoscleral junction, and circular fibers that are sphincter-like around the eye. 3. Ciliary muscle contractionâcauses the eyeball to become narrower and thus decreases tension on the lens, allowing it to become more spherical. When the ciliary muscle relaxes, the lens is pulled flat. The ciliary muscle is innervated by parasympathetic nerves and serves to increase the eye’s refractive power for accommodation to focus on closer objects. 4. PupilâWhen the pupil is small, the eye’s focus is better because most of the light enters at a fairly straight trajectory and less refraction is needed. 5. Eye fluidâmaintains distention of the eye a. Vitreous humorâa gelatinous mass held by a fibrillary network of proteoglycans. It does not flow, but some diffusion occurs. b. Aqueous humorâfree-flowing fluid. A balance of production and reabsorption regulates intraocular pressure. The ciliary body’s ciliary processes secrete aqueous humor by active Na+ secretion that draws in Clâ, 6. Layers of the eyeâsclera, choroid (vascular layer), and retina 7. Retinaâcomposed of outer-pigmented layer, rods and cones, outer limiting membrane, outer nuclear layer (containing cell bodies of the rods and cones), outer plexiform layer, inner nuclear layer, inner plexiform layer, ganglionic layer, optic nerve fiber layer, and inner limiting membrane. The outer pigment layer contains black melanin to prevent light reflection and stores large quantities of vitamin A for exchange with the rods and cones. It is absent in albinism, causing those affected to have poor visual acuity. 8. Retinal blood supplyâThe inner layers are supplied by the central retinal artery that enters through the optic nerve; the outer layers are adherent to the choroid and are supplied by diffusion from the choroid to the outer segments of the rods and cones. 9. Maculaâthe region of the retina with the highest visual acuity, measuring 61 mm2 10. Foveaâthe center of the macula that contains only cones, with the inner layers set aside to provide improved vision 11. Blind spotâ15 degrees lateral to central vision, due to the medial location of the optic disk in the retina 12. Rods and conesâRods have outer segments containing light-sensitive rhodopsin with transmembrane proteins and multiple membrane folds shaped into disks. Cones contain other types of photo-chemicals. Cones are 300 times less sensitive than rods, so color vision is poor in dim lighting. The rod pathway connects to bipolar cells, then to amacrine cells, and finally to ganglion cells. The cone pathway, however, is phylogenetically newer, faster, and uses larger cells and fibers; the cone pathway connects to bipolar cells and then to ganglion cells. The neurotransmitter of rods and cones is gluta-mate. Amacrine cells have at least eight types of neurotransmitters; all of them are inhibitory. 13. Innervation of the eye a. Parasympathetic innervationâbegins at the EdingerâWestphal nucleus, transmitting via the third nerve â ciliary ganglion behind the eye â short ciliary nerves â ciliary muscle (for accommodation) and iris sphincter (for miosis) b. Sympathetic innervationsâfrom the T1 level â sympathetic chain â superior cervical ganglion, up along the carotid artery to the small vessels, and then as the long and short ciliary nerves to the eye’s radial iris fibers (for mydriasis), to the MĂźller muscle of the eyelid and weakly to the ciliary muscle c. Accommodationâfrom Brodmann areas 18/19 to the pretectal/EdingerâWestphal area, and on to the ciliary muscle. The accommodation reflex elicits slight pupillary constriction. If there is no response to light, yet the accommodation reflex is intact, this is called an ArgyllâRobertson pupil (as seen with syphilis). d. The pupil is innervated at the iris sphincter by parasympathetic nerves, and at the radial iris muscles by sympathetic nerves. The light reflex is activated by impulses from the retina â optic tract â pretectal nucleus â EdingerâWestphal nucleus â third nerve â iris sphincter. It is decreased in syphilis, ethanol intoxication, etc. C. Visual conditions 1. Presbyopiaâcaused by lack of accommodation as the lens becomes larger, thicker, and less elastic with advancing age, causing the eye to remain permanently focused at a certain distance. Bifocals are required for near and far clarity. At 45 years of age, only 2 D of change is present; at 70 years, usually no change is present. 2. Hyperopia (farsightedness)âcaused by either a short eyeball or a weak lens that does not curve sufficiently when the ciliary body is relaxed (i.e., the ciliary muscle is contracted). Near vision is difficult. This condition is treated with a convex lens. 3. Myopia (nearsightedness)âcaused by an eyeball that is too long or a lens that is too strong. Light focuses in front of the retina because the lens is unable to straighten sufficiently despite a relaxed ciliary muscle. Far vision is difficult. This condition is treated with divergence of rays by a concave, spherical lens. 4. Astigmatismâcaused by refractive error of the lens system from an oblong cornea (rarely from the lens). Rays focus at one distance in one plane, and another in another plane. It is treated with spherical and cylindrical lenses of a certain axis. 5. Cataractsâopacities of the lens due to build-up of coagulated, denatured protein. They are treated by removal of the lens. 6. Glaucomaâincreased intraocular pressure; levels of 60â70 mm Hg commonly cause blindness, although even levels slightly > 20 mm Hg may compromise vision. The elevated pressure on the optic disk causes atrophy. It is caused by decreased trabecular aqueous humor outflow, as occurs in acute inflammation or chronic fibrosis. It is treated with drops to decrease aqueous humor formation or to increase its absorption. It may also be treated surgically to open the outflow pathway. 8. Scotomaâan area of decreased vision surrounded by preserved vision in the visual field. Causative etiologies include lead, tobacco, retinal disease, glaucoma, macular degeneration, retinal ischemia, and trauma. 9. Strabismus (cross-eyed)âwhen eye fusion mechanisms are not coordinated. It may be horizontal, vertical, or torsional (rotational). One eye may eventually become suppressed, leading to decreased acuity during development. 10. Horner syndromeâcaused by impaired sympathetic input resulting in miosis, ptosis (i.e., sympathetic nerves innervate the smooth muscle of the eyelid), anhidrosis, enophthalmos, and dilated facial vessels D. Photochemistry 1. Important molecules a. Rhodopsinâlight-sensitive pigment; combination of scotopsin + 11-cis-retinal. When light energy is absorbed, rhodopsin decomposes via photoactivation of an electron in the retinal component, converting it to the trans form, which pulls away from scotopsin (i.e., rhodopsin + light â bathorhodopsin â lumirhodopsin â metarhodopsin-1 â metarhodopsin-2 â scotopsin and alltrans-retinal). Rhodopsin is reformed by all-trans-retinal â 2-cis-retinal + scotopsin â rhodopsin; it is also reformed by all-trans-retinal â all-trans-retinol (vitamin A) â 2-cis-retinol â 2-cis-retinal â rhodopsin. b. G proteinâEach G protein is regulated by many receptors and itself regulates many effectors. The Îą-subunit binds guanosine triphosphate (GTP); the βâ and Îł-subunits hold the Îą-subunit to the plasma membrane and modulate GTP/guanosine diphosphate (GDP) exchange. The conversion from GTP to GDP inactivates the G protein. β and Îł subunits stabilize GDP binding and inhibit the binding of GTP to inactivate the G protein. When it is activated, the Îą-subunit has decreased affinity for the β- and Îł-subunits. Activation of the G protein inhibits others in the membrane. c. Vitamin A (all-trans-retinol)âcontained in the cytoplasm of rods and the pigment layer of the retina. Retinal (a light-sensitive pigment) can be converted to retinol (i.e., vitamin A) for storage. 2. Phototransductionâoccurs as activated rhodopsin (i.e., metarhodopsin-2) initiates a G protein-mediated pathway, wherein cGMP phosphodiesterase converts cGMP to 5’GMP (thus decreasing the concentration of cGMP). This decreases current through cGMP-activated Na+ channels, leading to hyperpolarization. The resting membrane potential is â40 mV because the outer portion is leaky to Na+, whereas the inner segment actively pumps it out. The activated membrane potential is â80 mV, at maximal light intensity. Light only has to activate the receptor for 0.0001 milliseconds, but the receptor potential persists for longer than 1 second. The receptor potential is proportional to light intensity. There is an amplifying cascade effect, such that one photon of light causes the movement of millions of Na+ ions. Rhodopsin kinase inactivates rhodopsin within a fraction of a second. 3. Night blindnessâdue to a severe vitamin A deficiency, leading to inadequate amounts of photosensitive pigment to detect light in dim/dark settings. This may be cured within an hour with intravenous vitamin A.
I. Cellular Molecular Transport
II. Membrane Potentials and Action Potentials
III. Synapses and Neurotransmitters
IV. Sensory Receptors
V. Nerve Transmission
VI. Somatic Sensations
VII. Vision
, and H2O. Aqueous humor flows out of the pupil through small trabeculae at the irisâcorneal junction and through the Schlemm’s canal into the venous system.
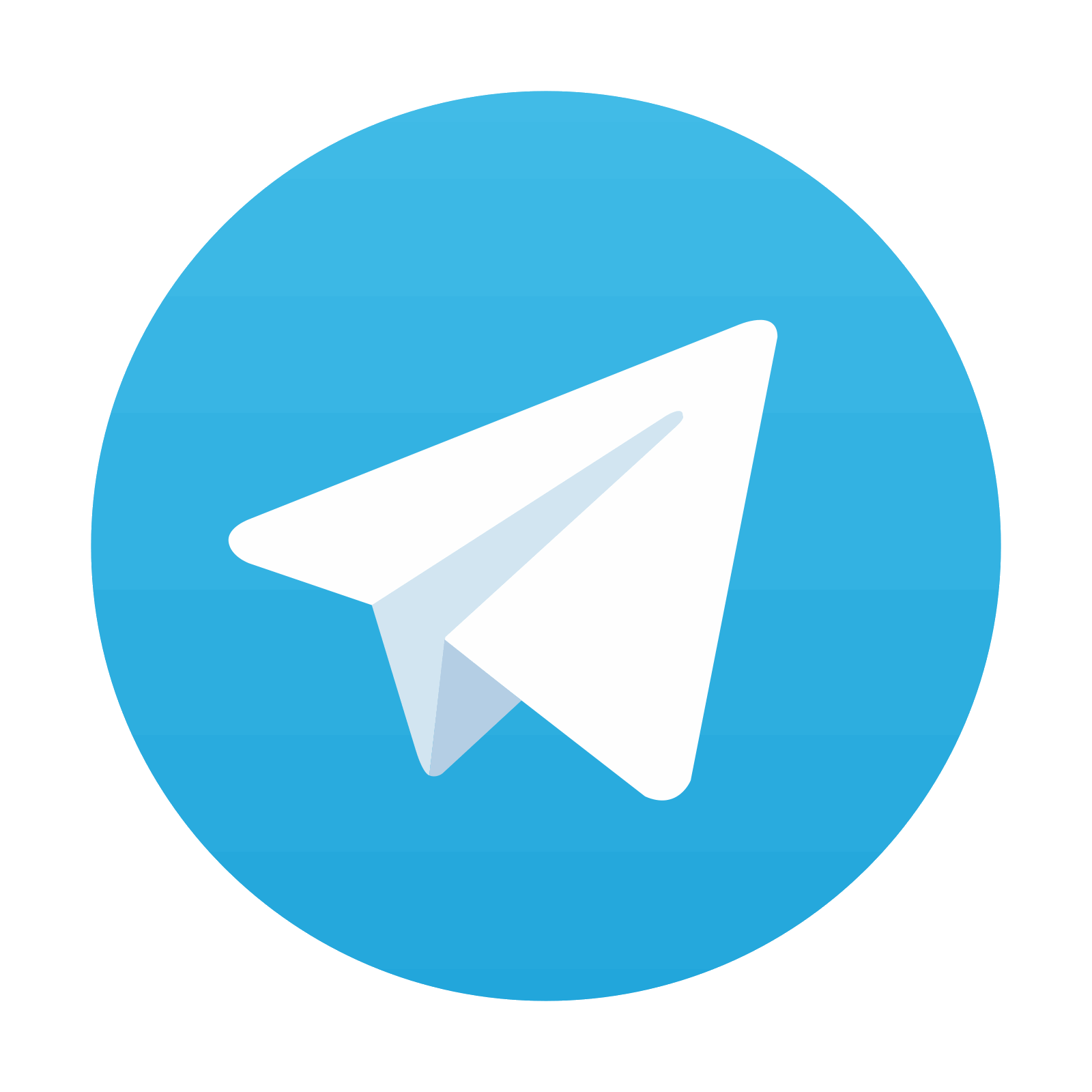
Stay updated, free articles. Join our Telegram channel
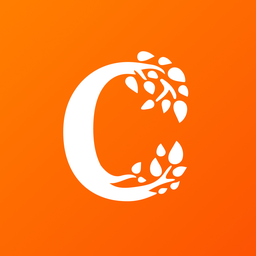
Full access? Get Clinical Tree
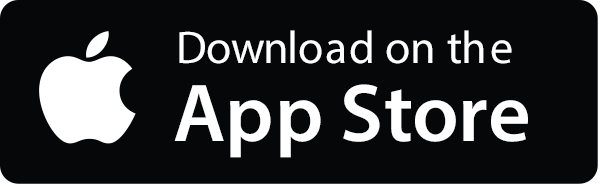
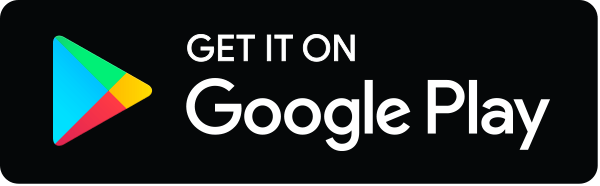