3 Physiology and Hemodynamics of Arteriovenous Malformations and Arteriovenous Fistulas
Abstract
Most studies on arteriovenous malformations (AVMs) and dural arteriovenous fistulas (AVFs; previously often called dural AVMs in older literature) have focused on the natural history, anatomy, histopathology, treatment, and prognosis of these lesions. More recently, studies have also been published on molecular development. However, few publications clearly describe the physiology and flow hemodynamics of these lesions. This chapter focuses on a summary of the historical and current knowledge and literature on the physiology and flow dynamics of AVMs and AVFs.
Keywords: arteriovenous fistula, arteriovenous malformation, autoregulation, flow hemodynamics, physiology, steal, venous hypertension
Key Points
- Arteriovenous malformations (AVMs) and arteriovenous fistulas (AVFs) are formed when an abnormally low-resistance pathway allows blood to be shunted from the arterial system to the venous system.
- In AVMs, arterial steal can cause chronic local tissue ischemia in areas surrounding the nidus due to shunting of blood through a low-resistance circuit, but this effect is not often seen in AVFs.
- In both AVMs and AVFs, venous hypertension and congestion can further contribute to surrounding tissue ischemia and increased risk of spontaneous hemorrhage or neurological dysfunction, such as seizure.
- It is theorized that normal perfusion pressure breakthrough, occlusive hyperemia, and loss of autoregulation contribute to postoperative edema and hemorrhage in patients after AVM resection.
3.1 Introduction
This chapter focuses on the physiology and hemodynamics of intracranial arteriovenous malformations (AVMs) and dural arteriovenous fistulas (AVFs, sometimes also called dural AVMs in older literature). These pathologically important lesions are characterized by direct arteriovenous (AV) shunts. AVMs are characterized by a nidus—a tangle of blood vessels in which the arteries and veins connect directly, without an intervening capillary bed. In contrast, AVFs may have many associated dilated pathological vessels, but the true pathology lies in the direct connection point or points. Until recently, few studies have been published regarding the flow and hemodynamics of cerebral AVMs and AVFs. However, in the last 10 years, there has been a significant increase in publication on this subject, leading to a better characterization of the physiology of AVMs and AVFs that can aid in our understanding and treatment of these lesions.
3.2 Normal Flow and Physiology
3.2.1 Overview
Models of normal flow through the human circulatory system (and subsequently cerebral circulation) have been created using adaptations of Ohm’s law and Poiseuille’s law. Poiseuille’s law applies to ideal newtonian fluids undergoing laminar flow, which occurs when blood flows at a steady rate through a long, smooth vessel, and the centermost portion of blood stays in the center of the blood vessel. Generally, these principles state that flow can be equated to the change in pressure for any given resistance, and flow rate is related to the change in pressure multiplied by πr4 and divided by the viscosity (or resistance) of the fluid and the length of the tube. Ohm’s law can be expressed by the equation
where Q is flow, ΔΡ is the difference in pressure between two measured points, and R is resistance. Poiseuille’s law can be expressed by the equation
where Q is flow, ΔΡ is the difference in pressure between two measured points, r is the radius of the tube, η is the viscosity of the fluid, and L is the length of the tube.
It should be noted that even small increases in vessel radius or diameter cause corresponding exponential increases in flow.
In normal circulation, however, blood is not a newtonian fluid, blood vessels are not rigid, and blood flow is not perfectly laminar. Many conditions, such as vascular stenosis, the presence of aneurysms, and asymmetry, can lead to turbulent flow, in which blood flows in all directions in the vessel and is continually mixing, in contrast to laminar flow. The occurrence of turbulent flow increases with increasing blood flow velocity, increasing diameter of the blood vessel, and increasing blood density. With respect to AVMs and AVFs, the factors above make exact modeling difficult. However, these basic principles form the foundation for understanding their hemodynamics.
3.2.2 Vascular Reactivity
Blood vessels have the ability to change vascular resistance in response to changes in arterial pressure in order to maintain a relatively constant rate of blood flow. This process allows the autoregulation of blood flow. In normal tissue with intact autoregulation, increases in arterial pressure cause a compensatory increase in vascular resistance through activation of local control mechanisms. Similarly, a decrease in arterial pressure causes a decrease of vascular resistance in order to maintain a stable rate of blood flow.1 In the brain, autoregulation protects tissues from experiencing ischemia or hyperemia within a range of blood pressures.2
The processes by which this autoregulation occurs, arterial vasodilation and vasoconstriction, have many biochemical mechanisms. In a hallmark study, Furchgott and Vanhoutte3 reviewed the importance of endothelium-derived relaxing factor (EDRF), which stimulates a cyclic guanosine monophosphate-mediated relaxation mechanism. Increased wall shear stress, which can be observed in increased arterial pressure or turbulent flow, stimulates the release of EDRF. In laboratory studies, increased flow induces vasodilation, which is not observed when the endothelium is removed. Furchgott and Vanhoutte also showed that endothelium-derived contracting factors, such as arachidonic acid, augment arterial contractions evoked by norepinephrine, and these findings were also not observed after removal of the arterial intima.3
3.3 Arteriovenous Malformations
3.3.1 Overview
In AVMs, an abnormal connection exists between the arterial and venous systems that bypasses the normal intervening network of capillaries. Although a clear genetic transmission pattern has not yet been defined, the occurrence of AVMs has been associated with some genetic syndromes, in particular, hereditary hemorrhagic telangiectasia.4 The AVM nidus is a point toward which multiple feeding arteries converge and from which an enlarged vein or veins drain.5 AVMs may drain through either the deep or the superficial venous systems, and although there may be large or dysplastic draining veins, the drainage generally continues in an anterograde fashion into the normal venous system. Conventional angiography is the preferred method for evaluating the anatomy of AVMs. Angiography clearly defines feeding arterial vessels, nidus location, venous drainage patterns, the presence of aneurysmal dilations, venous varices, and vasculopathy. Often, AVMs with retrograde leptomeningeal venous drainage have intrinsic varices or aneurysms associated with them that affect their hemodynamic stability and risk of hemorrhage. An increased risk of hemorrhage is conferred by small AVM size, high-flow lesions with increased intraluminal pressure, deep location, and previous AVM hemorrhage. Angiographic characteristics such as intranidal aneurysm, flow-related arterial aneurysm, deep venous drainage, and venous stenosis have also been associated with an increased risk of AVM hemorrhage.6,7 The overall annual risk of hemorrhage is approximately 2 to 4%, with higher risk for ruptured lesions than unruptured lesions.8,9
3.3.2 Physiology and Hemodynamics
AVMs lack a diffusing capillary bed and therefore are subject to high-flow shunting and increased wall shear stress forces through feeding arteries and intranidal vessels.10 This shunting can initiate abnormal vascular remodeling, which leads to dilatation of vessels and aneurysm formation. The low-resistance environment of the AVM causes a sump effect, drawing flow so that the adjacent brain tissue surrounding an AVM also experiences reductions in blood flow, which leads to a state of chronic hypoperfusion.11 The transfer of high-pressure arterial blood to the veins through this low-resistance circuit also results in venous hypertension and can generate aneurysms and promote venous mural remodeling, which especially in the setting of impaired outflow can produce rupture.12 An illustration of relative pressures in an AVM circuit are shown in ► Fig. 3.1a, with a pressure gradient that favors flow through the AVM and diverting flow from arterial territories downstream.
Fig. 3.1 Illustration of changes in relative pressure in AVM feeding vessels, AVM nidus, AVM draining vein, and normal veins. (a) At normal systemic pressure, relative pressures in arterial feeding vessels, AVM nidus, draining vein, and normal veins are high, medium, medium, and low, respectively. (b) Systemic pressure is decreased intraoperatively to decrease flow through the AVM. Relative pressures through arterial feeding vessels, draining vein, and normal veins are medium, low, and medium, respectively, and pressure in the AVM nidus is low because of disconnection of arterial feeders. (c) After the AVM nidus has been resected and the patient’s systemic pressure has returned to normal, the relative pressures in the arterial feeders and normal veins are high and low, respectively. When the feeding vessels experience a return to normal perfusion pressure, it is theorized that there is a loss of the ability to autoregulate, and there may be postoperative hyperemia or hemorrhage. (These images are provided courtesy of Barrow Neurological Institute, Phoenix, Arizona.)
Uranishi et al studied the expression of smoothelin, a cytoskeletal protein found in mature smooth muscle cells used as a marker for the identification of these particular cells.13 The authors examined vascular smooth muscle cell differentiation in various lesions, and found decreased expression of smoothelin in large AVM vessels when compared to vessels in the normal brain. They postulated that the decrease in smoothelin expression correlated with a reduced presence of mature vascular smooth muscle cells in AVMs and subsequent diminished autoregulation and contractility in AVM vasculature. The decrease in smoothelin expression was associated with increased hemodynamic stress within the AVM.13 Gao et al have published evidence that endothelial progenitor cells are present in increased levels in AVMs and that they contribute to the continuous pathological remodeling of the lesion.14
The prevailing theory in growth and rupture of AVMs involves inflammation and remodeling of the extracellular matrix. Evidence suggests that proinflammatory cytokine release, increased expression of cell adhesion molecules, and inflammatory cell invasion in the vessel walls contribute to the instability of the AVM nidus.12,15 Recent animal studies have shown a correlation between increasing wall shear stress and AVM angiogenesis.16 In high-flow AVMs, increasing diameter and variability of nidal vessels can cause turbulent flow, as evidenced by endothelial cell turnover, focal dilatation of vessels, and platelet aggregation.16
Imaging Techniques Used to Characterize Hemodynamic Parameters
Real-time indocyanine green videoangiography (ICG-VA) has been used to help identify vascular architecture, flow direction, and qualitative blood flow transit speed.11 FLOW 800 software has been applied by one group to obtain quantitative flow measurements from the operating microscope ICG-VA data.11 The data should be interpreted as relative quantitative values because they are based on an arbitrary intensity number to denote flow.11
Intraoperative micro-Doppler flowmetry has been used and validated in cerebral aneurysm surgery, and more recently has been used to model flow through cerebral AVMs.17,18 Metrics such as velocity (with direction), pulsatility index, and resistance index (RI) are useful for characterizing flow in AVMs. These metrics are obtained from arterial feeders, venous draining vessels, and relevant vessels during dissection or possible transit arteries.17,18 RI is defined by the equation
This value is calculated in real time by Doppler imaging and is used to distinguish AVM feeding arteries from normal arteries.
The RI is an important method for characterizing AVM flow intraoperatively, because it is not affected by the angulation of the Doppler probe, which reduces operator error in obtaining measurements. After surgical resection of an AVM, the RI of the feeding artery returns to values within the normal range.19
Flowmetry can be used to distinguish between arteries and veins by evaluating flow direction when ICG-VA is not conclusive, and it can also be used to identify multiple draining vessels and secondary draining veins. Main draining veins have a higher flow quantity, and secondary draining veins have a relatively lower flow quantity. Spetzler–Martin grade II AVMs have the highest arterial and venous flow rates. Flowmetry is of limited value for measuring small, deep vessels of AVMs because of the difficulty of positioning a probe on these vessels. Another disadvantage of using this technique is the potential for injuring fragile draining veins during the measurement process.18
Noninvasive quantitative magnetic resonance (MR) angiography has been used to characterize hemodynamic factors contributing to the formation of aneurysms in AVMs. The investigators examined flow, vessel diameter, and wall shear stress in AVM feeding vessels with and without aneurysms. A significantly greater degree of increased wall shear stress was seen in feeding arteries with aneurysms than in those without aneurysms.20 The same investigators found that successful AVM embolization and resection reduced wall shear stress in feeding vessels down to normal values.21
Vascular Steal
Vascular steal is thought to occur in AVMs because of the relatively low resistance to flow in the AVM vasculature compared to the circulation in neighboring tissue. This disparity creates a pressure differential that causes blood flow to be shunted away from neighboring brain tissue. Supporters of this theory believe that the steal phenomenon leads to a state of chronic hypoperfusion of neighboring brain tissue, contributing to brain dysfunction and loss of vascular autoregulation.22 Spetzler et al associated low feeding artery pressures measured in large AVMs to fluctuations in neurological deficits because of relative ischemia.23 One proposed mechanism of seizure associated with AVMs is related to vascular steal. It is thought that AV shunting away from the downstream arterial territory causes hypoperfusion-induced remodeling of the perinidal cortex, creating a molecular environment conducive to epileptogenesis.24 This theory is controversial, because many AVMs have low-flow states with no angiographic evidence of steal and no significant contribution of arterial supply from the internal carotid or vertebral arteries.25 Others have produced concordant results that show no significant difference in feeding artery pressure, arterial flow velocity, or pulsatility index between patients who present with focal neurological deficits and those who present without deficits.26 The exact pathophysiological mechanism of steal, if it exists, is unclear; however, it remains a useful concept.
CO2 Reactivity
Hypercapnia causes dilation of the arterioles and increased cerebral blood flow, and the reverse is true in hypocapnia. This effect has been studied using ventilatory control as the mechanism to affect CO2 regulation. Classically, in AVMs, it was thought that when hypercapnia is induced, feeding vessels show only a slight increase in flow, as measured by Doppler, and in hypocapnia feeding vessels show stable flow.27 In a later study by De Salles and Manchola, hypocapnia was shown to significantly decrease flow velocity in both AVM feeding and non-feeding vessels as measured by transcranial Doppler at the level of small vessels.28 Conversely, during hypercapnia, flow volume was significantly higher in AVM feeding vessels when compared to nonfeeding vessels.28 Of note, the definition of measured vessels was specific to this study, and transcranial Doppler is operator-dependent with regard to the validity of measurements. After resection of an AVM, a normal vasomotor response is observed, particularly in branches of vessels that supplied the AVM.27
Autoregulation and Normal Perfusion Pressure Breakthrough
There are conflicting reports on whether autoregulation is preserved in tissues surrounding AVMs; one group reports augmented vasodilation, but others show intact autoregulation pre- and postoperatively. Hoffman et al noted that the tissue surrounding an AVM has lower partial pressure of oxygen (Po2) at baseline, with a normal partial pressure of carbon dioxide (Pco2) and pH. In this study, induced hypercapnia caused an increased Po2 and a decreased pH in the surrounding brain tissue with no change in Pco2. Hypercapnia was thought to transiently increase cerebral blood flow and wash out local CO2 in the tissues surrounding the AVM.29
Intraoperatively, systemic arterial pressure is decreased and arterial feeding branches are disconnected from the AVM nidus (► Fig. 3.1b). Spetzler et al defined the term “normal perfusion pressure breakthrough,” in a theory that stated that impaired autoregulation contributes to the formation of AVMs, and to edema and hemorrhage after AVM resection (► Fig. 3.1c).30 The theory stated that because of chronic hypoperfusion of the tissue surrounding an AVM, vascular CO2 reactivity and autoregulation were lost. Larger AVMs have a lower flow resistance because of larger feeding vessels. After AVM resection, redirection of blood flow to chronically dilated vessels that have lost the ability to vasoconstrict at the level of resistance vessels overwhelms their capacity, resulting in edema or hemorrhage.30 In an updated review of the literature regarding this theory, Rangel-Castilla et al found that postoperative hyperventilation, hyperoxia, and nitric oxide balance play a role in restoring normal autoregulation.2
Young et al showed that, in areas of normal brain supplied by AVM feeders, when systemic arterial pressure increased, there was no resultant increase in cerebral blood flow.31 The authors postulated that chronic hypotension in areas that are supplied by AVM feeding vessels may be associated with an adaptive shift or displacement of the intact autoregulation curve to the left, but it is not associated with loss of autoregulation or “vasomotor paralysis.” They also reported no instances of postoperative edema or hemorrhage; however, they stated that an increase in pressure postoperatively that exceeded the upper limit of the autoregulatory curve could cause “breakthrough” edema and hemorrhage.31
Schaller et al pointed out that a difficulty in measuring cerebral vascular reactivity (CVR) is that it cannot be measured quantitatively with adequate temporal and spatial resolution.32 These authors employed the use of spectrophotometry to compare AVM-specific CVR patterns with a control group, and found no clinically significant difference between the two groups before or after surgery.32
Venous Hypertension
AVM draining veins are often dilated from experiencing increased blood flow from AV shunting, which can result in variceal or aneurysmal formation. Wall shear stress can play a role in rupture by promoting remodeling that causes decreased integrity of vessel walls and weakening of draining veins. However, an inverse relationship exists between the number of draining veins and venous outflow pressure; a greater number of draining vessels decreases the venous pressure in the draining system, because of more potential outflow pathways for the AV shunted blood. Increased intravascular pressure may lead to tissue hypoxia and ischemia upstream from the nidus, and venous congestion also impairs outflow from surrounding brain tissue that drains to the same venous bed as the AVM. If the draining veins are obstructed, increased pressure can open preexisting AV channels and further contribute to the growth of AVMs.33
Increased venous outflow caused by AV shunting can result in venous congestion and may impair microvascular autoregulation. This mechanism of venous congestion is thought to result in parenchymal irritation and subsequent epileptogenesis.34
A proposed mechanism termed “occlusive hyperemia” was discussed by al-Rodhan et al in 1993.35 They described a mechanism in which stagnation of arterial flow in AVM feeding vessels, exacerbated ischemia of surrounding tissue, and obstruction of draining veins caused venous engorgement, hyperemia, and worsening stagnation of arterial flow. These factors contributed to an environment prone to hemorrhage and edema after AVM resection,35 and can cause postoperative neurological deficits.
3.4 Arteriovenous Fistulas
3.4.1 Overview
AVFs are characterized by abnormal connections between the arterial and venous systems with no true nidus as seen in AVMs.36 They have been associated with a history of trauma, prior surgery, and congenital abnormalities, which lead to venous sinus thrombosis and other factors that contribute to the formation and progression of AVFs (► Fig. 3.2).7,37 The arterial supply of AVFs is typically recruited from dural arteries and from meningeal branches of cerebral arteries. The venous drainage occurs through dural venous sinuses or through other dural and leptomeningeal venous outflow tracts.37 They do not contain a “nidus” in the parenchyma, but do contain one or more AV shunts with relation to the dural vasculature.38 Intracranial AVFs are classified according to different grading scales, including the traditional Borden-Shucart system,39 the Cognard system,40 and the UCSF41 system. These grading systems typically characterize AVFs by the type of venous drainage present in the lesion. AVFs are most often observed at the transverse, sigmoid, and cavernous sinuses.42 As seen with AVMs, AVFs may exhibit retrograde leptomeningeal venous drainage, and additionally can be associated with varices and aneurysmal dilation which, when present, increase the risk of hemorrhage. Angiographic evidence shows that variceal or aneurysmal formation, retrograde leptomeningeal venous drainage, and galenic venous drainage are all associated with increased risk of hemorrhage.37 Hemorrhage risk in patients with AVFs associated with retrograde leptomeningeal venous drainage is approximately 8.1%, with a mortality rate of 10.4% annually.25
Fig. 3.2 Factors contributing to the formation and progression of dural AVFs. Trauma, surgery, and congenital factors contribute to venous sinus thrombosis, which along with other factors has been associated with dural AVF formation. Recruitment of arterial feeders into the AV communication leads to shunting (*), neighborhood symptoms such as pulsatile tinnitus and cavernous sinus syndrome (‡), and an increase in local and generalized venous pressures, resulting in nonhemorrhagic focal neurological deficit. Prolonged increase in venous pressure leads to retrograde leptomeningeal venous drainage and the formation of venous varices and aneurysms, which are prone to hemorrhage and rupture. Generalized increases in venous pressure, and additional venous varix or aneurysm formation, can cause aqueductal obstruction and further contribute to increased intracranial pressure. Most dural AVF are asymptomatic and spontaneously thrombose (†) without further recurrence. (Adapted from Awad et al.37)
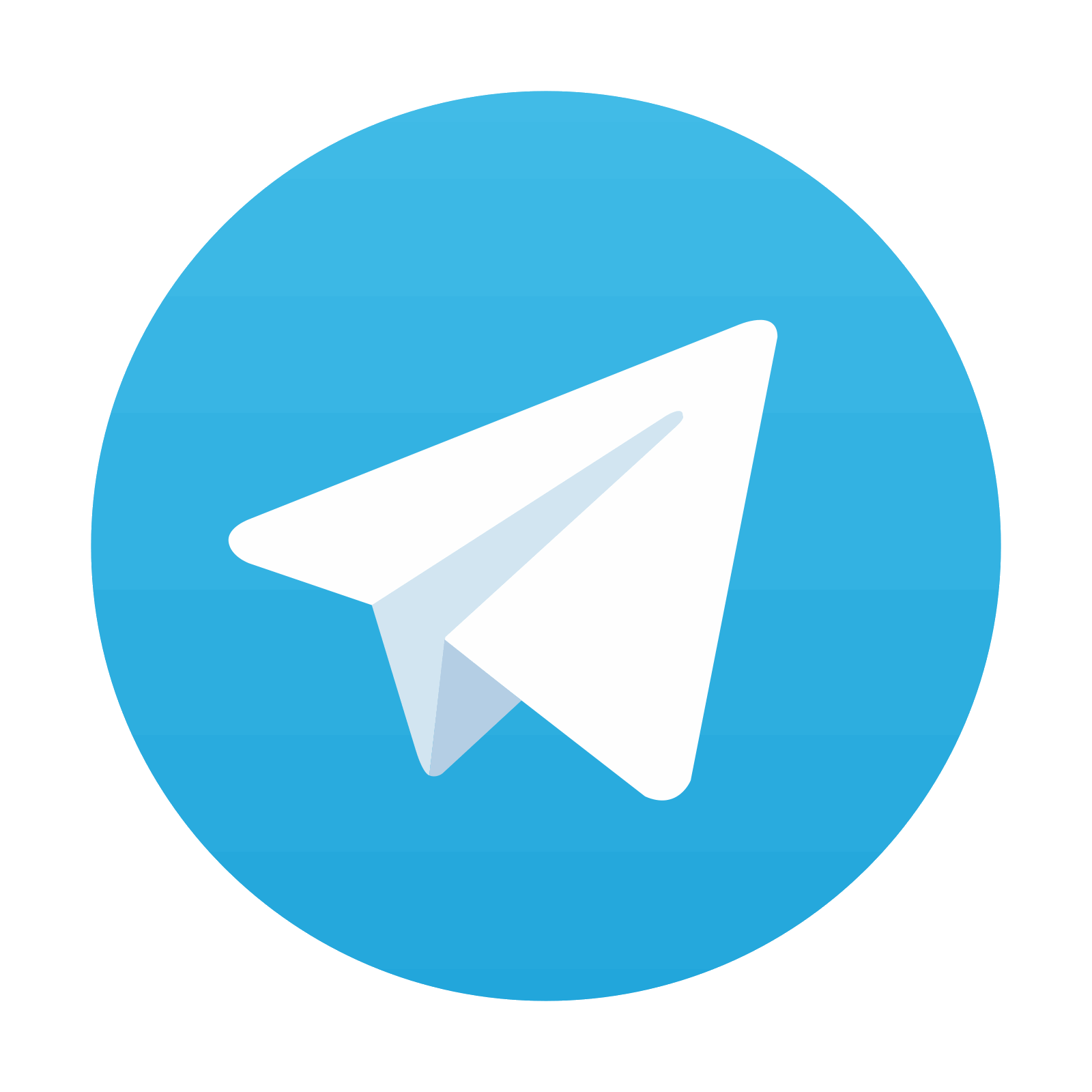
Stay updated, free articles. Join our Telegram channel
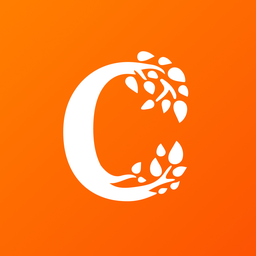
Full access? Get Clinical Tree
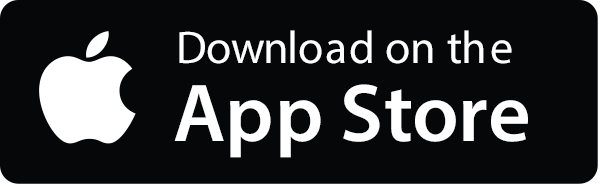
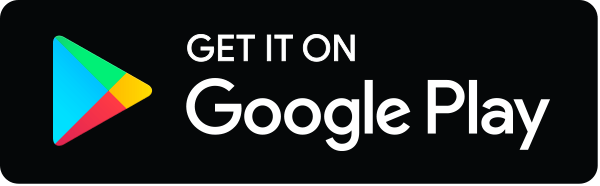