Overview
Over the past several decades the field of neuromodulation has seen a rapid growth. This growth has been possible in part by the many advances made in technology; in particular the improvements that have been made in power sources for implantable products. The following chapter examines the diverse power requirements necessary to treat the many indications currently targeted for neuromodulation therapy and how improvements in technology have allowed the expansion of neuromodulation into more and more complex disorders. A comparison of the different types of power sources and the advantages and disadvantages of each will be discussed taking into consideration patient compliance and costs. Finally, this chapter will end by briefly looking at some of the new power sources that are currently in the research stage and how these may affect neuromodulation in the future.
Power requirements
The development of any new neuromodulation device first begins by examining where and how the device is intended to be used. Several questions need to be answered to allow the engineer to design a device capable of supplying the appropriate output to treat the desired disease. These questions include: what are the energy requirements necessary to provide a therapeutic result? Where will the devices be implanted? And what type of interaction will there be between the devices? The answers to these and other questions will help the designer understand the complexity of the device that is needed, the size of the device, and the materials needed to construct the device. Finally, all of these attributes need to be balanced with the overall cost of the device.
Therapeutic energy requirements
An important factor that needs to be addressed early in any design is the capacity and type of power source. There is the obvious need to keep the overall size of any medical device as small as possible, however, this must be balanced with the requirement to provide the necessary complexity and output parameters in order to provide the appropriate therapy to the patient. To date, there have been a number of implantable devices developed to treat a variety of medical conditions with each of these conditions having unique power requirements.
Figure 11.1 demonstrates the energy requirements (energy usage) and the complexity (number of contacts) needed to treat some of the currently approved indications. Energy requirements can be compared using therapeutic average current (ITAVG), which is calculated from:
ITAVG = FREQ * PW * AMP

The highest energy requirements and complexity for currently approved indications are in spinal cord stimulation (SCS). Parameter selection for SCS involves selecting the optimal electrode configuration followed by adjusting the amplitude, width and frequency of electrical pulses . The choice of electrodes depends on the extent of the pain. Systems today can control up to 16 independently programmed electrodes using multiple stimulation programs running sequentially. Stimulation amplitude is measured in either milliamperes or Volts and is a measure of the intensity of stimulation. This is set within a range of 0–25 mA (1–10 V) according to the type of electrode used and the type of nerves stimulated. Lower voltage is chosen for peripheral nerves and paddle type electrodes. Pulsewidth usually varies from 100 to 400 μs. Pulse frequency is measured in Hertz (Hz) and usually delivered between 20 and 120 Hz . Power sources for SCS devices are usually in the range of 2–7 Ah. These high energy requirements are necessary due to the variety of painful conditions and the relatively large distance the current must travel to reach the target nerve fibers. Some of the indications currently being treated with SCS include failed back surgery syndrome, peripheral neuropathy, complex regional pain syndrome, angina pectoris, pain due to peripheral vascular disease, and stump pain . Spinal cord stimulation leads are implanted into the epidural space above the spinal cord. They produce their effect by activating fibers below located in the dorsal columns of the spinal cord. Therefore, the electrical field must travel through the epidural tissue, through the dura, through the cerebral spinal fluid, and finally to the dorsal columns. Aside from the actual distance, which can be up to several millimeters, each of these areas has a particular electrical conductance which impedes the flow of current or shunts the field away from the intended target . It has been theorized that only 10% of the current that leaves the electrode actually makes it to the target nerves . On the opposite end of the range are deep brain stimulation (DBS) and cardiac pacemakers. Electrodes implanted into the brain are in direct contact with the target nerve fibers. Therefore, the electrical field needed to activate deep brain targets is much smaller. The therapeutic parameter used for DBS range between 1 and 3 mA amplitudes, 60–210 μs pulsewidths and 100–130 Hz frequencies . Similarly, cardiac pacemakers have a relatively low battery capacity (1–1.5 Ah).
Since neurostimulation is fundamentally delivering small doses of electricity, the system must provide a path through the patient for the electricity to flow and the complex form of Ohm’s Law, V = I * Z, can be used to provide a quantitative description of the requirements for the power source. The law states that voltage, V, is equal to the product of current, I, and the complex impedance, Z. The power source capacity is frequently given in units of ampere-hours using the symbol Ah. Therefore, given the desired expected life of the power source you can calculate the maximum average current allowed for the neuromodulation device to deliver therapy. We will consider other contributing factors to the maximum average current (IMAVG) in the System Power Requirements section.
System power requirements
The efficiency of the system being used to deliver the desired therapeutic effect has an impact on the overall power requirements. Figure 11.2 shows a general block diagram for the elements of the system that are in series, including a power source, voltage conversion, pulse generator electronics, delivery mechanism, patient interface and patient.

Power source
The power source, as the name implies, is the source of electrical energy for the system. There are a wide range of voltages, capacities and other characteristics that depend on the type chosen. Details on options for this element of the system are covered later in this chapter.
Voltage conversion
Now let us consider the contributions of the system elements to the overall power requirements using Ohm’s Law, V = I * Z, simplification. The electronics and power source must create the voltage (V) necessary to overcome the impedance (Z) presented by the patient, patient interface and delivery mechanism combined in series in order to deliver the therapeutic current (I TAVG ). This often requires circuitry in the electronics to multiply the voltage of the power source, resulting in a multiplier (M) times the power source voltage.
Pulse generator electronics
In general, the electronics are responsible for converting the raw energy from the power source into the desired stimulation waveforms, monitoring important signals and enabling communication with external patient or clinician devices. The added complexity needed to treat some neuromodulation modalities requires highly advanced electronics. It remains important that electronics used in the design of implantable devices is efficient with respect to its energy consumption and size.
Delivery mechanism
The delivery mechanism is a mechanical connection that conducts the electrical pulses from the stimulator to electrodes at the patient interface. The electrical resistance of the connection depends upon the construction, materials and the length of the connection. The length is highly dependent upon implant location relative to the neurological target. There are systems that integrate the delivery mechanism with the stimulator, such as the BION ® microstimulator, where the length would be less than 1 cm. On the other hand, a stimulator placed in the upper buttock that had a patient interface in the cervical region could be in the range of 90–110 cm.
Patient interface
Electrical stimulation and recording of excitable tissue is the basis of electrophysiological research and clinical functional electrical stimulation, including deep brain stimulation and stimulation of muscles, peripheral nerves or sensory systems. When a metal electrode is placed inside a physiological medium, such as extracellular fluid (ECF), an interface is formed between the two phases. In the metal electrode phase and in attached electrical circuits, charge is carried by electrons. In the physiological medium, or in more general electrochemical terms the electrolyte, charge is carried by ions, including sodium, potassium, and chloride in the ECF. The central process that occurs at the electrode–electrolyte interface is a transduction of charge carriers from electrons in the metal electrode to ions in the electrolyte. The patient interface is time-varying impedance. Figure 11.3 illustrates a simple electrical circuit model of the electrode–electrolyte interface, consisting of two elements . C dl is the double layer capacitance, representing the ability of the electrode to cause charge flow in the electrolyte without electron transfer. Z faradaic is the faradaic impedance, representing the faradaic processes of reduction and oxidation where electron transfer occurs between the electrode and electrolyte. One may generally think of the capacitance as representing charge storage, and the faradaic impedance as representing charge dissipation .

It is important that these faradaic reactions are reversible. The electrode material, electrode surface area, maximum charge per phase and charge balance are all considerations for safe pulse delivery. DC blocking capacitors are generally used in the pulse generating electronics to couple pulses to the stimulating electrodes to prevent DC current from generating harmful faradaic reactions.
Patient
The patient adds a tissue conductance to the system that varies by implant location. For SCS, when the electrodes are implanted in the cervical region, the typical distance between the electrode surface and the dorsal columns is very close as measured by the threshold of activation compared to electrodes implanted in the thoracic region where threshold can be very high. The impedance of the patient is determined by both the conductance of the tissue ( ρ ) and the geometrical surface area of the stimulating electrodes (a) as given in the following formula:
Z = ρ 2 p a
he smaller the electrode area for a given tissue conductance the higher the impedance .
Neuromodulation devices exist that provide current, voltage and charge controlled therapy. In order to keep the formula in this chapter more straightforward, we will use the controlled current convention. Conversions may be made to the other conventions using well documented relationships from the physics of electricity.
Now let us consider the contributions of the system elements to the overall Impedance (Z):
Z T o t = Z E l e c + Z D M + Z P I + Z P
The total impedance Z Tot is equal to the pulse generator electronics impedance Z Elec plus the distribution mechanism impedance Z DM plus the patient interface impedance Z PI plus the patient electrode tissue impedance Z P . Thus:
V = I * Z T o t
V is the neuromodulation system voltage requirements to deliver a therapeutic pulse of amplitude I. We now can calculate the required system power source capacity:
C = I A v g * T L
The power source capacity C is equal to the average current consumed from the power source times the therapeutic life time in hours.
The formula for average current (I AVG ) measured in amperes:
I AVG = ( M * I TAVG ) + I EAVG
M = V P S V > V P S
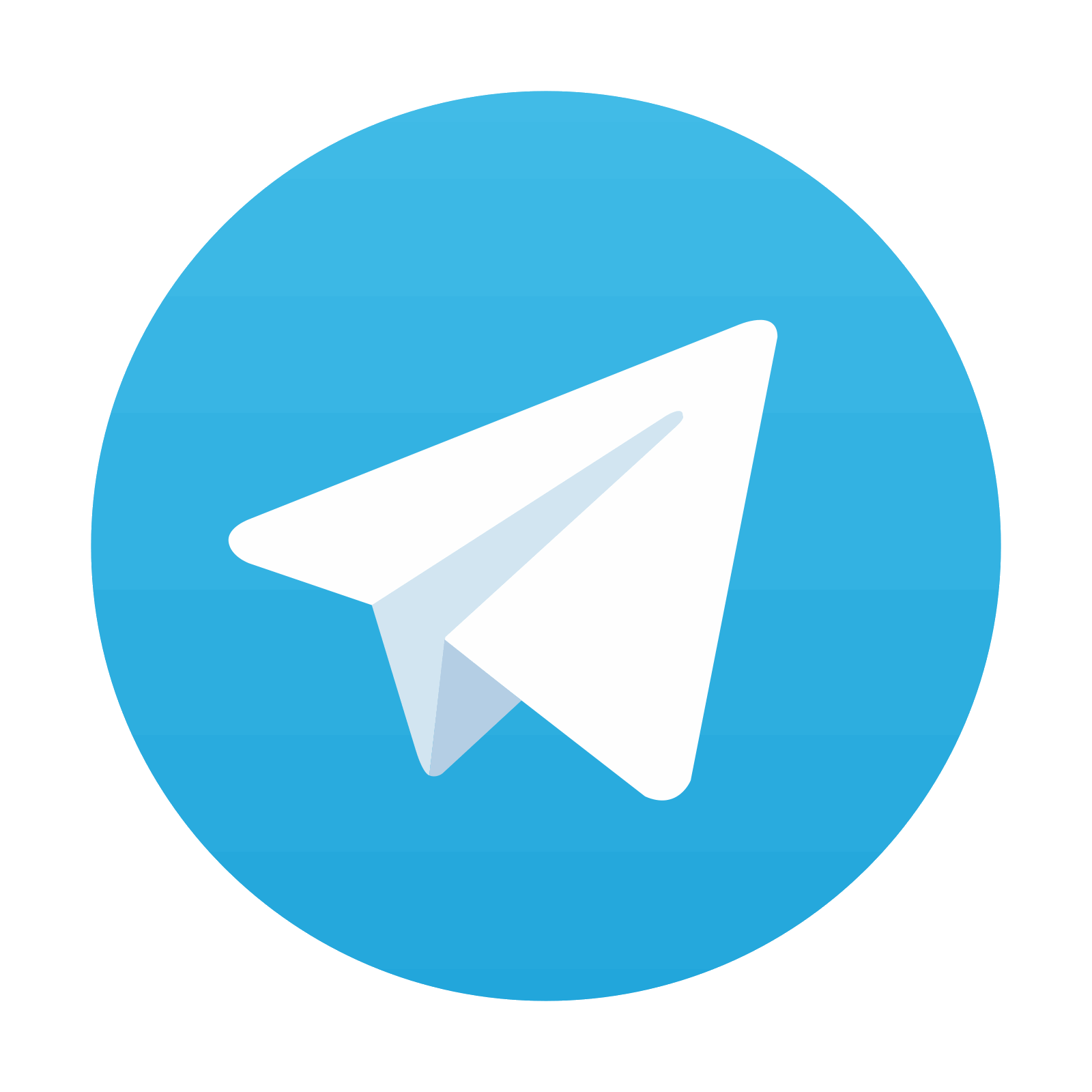
Stay updated, free articles. Join our Telegram channel
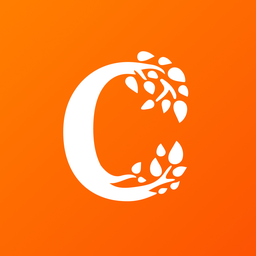
Full access? Get Clinical Tree
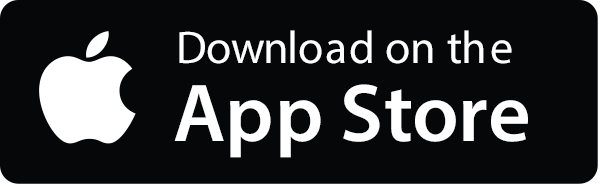
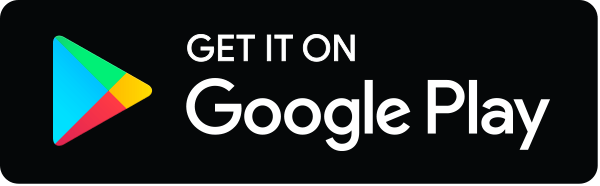