Summary of Key Points
- •
An overview of the anatomic aspects of the human spinal column is presented.
- •
Geometric features of the vertebrae, intervertebral discs, ligaments, facet joints, and muscles are given.
- •
Interconnectivity of these components and their compositions are addressed.
- •
Some basic concepts and biomechanical terminologies (motions describing kinematics and loads describing forces and bending moments in different planes) are introduced with a focus on normal day-to-day, physiologic and traumatic spine responses.
- •
Concept of injury initiation based on the four phases in the nonlinear response (force-deflection) is presented.
- •
Role of individual structural components of the spinal column in maintaining its stability and describing the clinical biomechanical aspects is discussed.
- •
Properties (e.g., failure loads, deflection, stiffness, energy) and geometric quantifications (e.g., length and area of ligaments) for various components at different regions are described along with numeric data.
- •
Regional biomechanical characteristics of the spine are described along with definitions of clinical instability.
- •
Anomalous anatomy is presented.
- •
Effects of sex (gender) and other variables such as loading/strain rate in modulating biomechanical responses are described.
Vertebral Column
The human spinal column consists of 33 vertebrae interconnected by intervertebral discs, facet capsules, and ligaments. Normally, there are 7 cervical (C1-7), 12 thoracic (T1-12), 5 lumbar (L1-5), 5 fused sacral (S1-5), and 4 separate coccygeal bones. The first three regions are flexible. The most common variations include sacralization of the fifth lumbar vertebra or lumbarization of the first sacral vertebra. Ventral and dorsal views of the spinal column with the skull are shown in Figure 7-1 . The normal adult vertebral column has four curvatures. The cervical and lumbar regions are lordotic, and the thoracic and lumbosacral regions are kyphotic. The lordotic curvature is convex ventrally, and the kyphotic curvature is concave ventrally. The thoracic and lumbosacral kyphotic curvatures exist in utero and are called the primary curvatures. The cervical and lumbar lordotic curvatures develop with the raising of the head postnatally and the assumption of the erect posture. The cervical curvature is shallow; it begins at the dens of the axis and terminates at T2. The lumbar lordosis develops due to the upright position of the trunk. The sacral curvature is relatively smooth and concave. Variations in the disc and vertebral body dimensions form and maintain these curvatures; they are often modified by age-related changes of the vertebrae, osteophyte development, trauma, congenital malformations, neurologic disorders, and imbalances of the paraspinal muscles. The center of gravity of the spinal column generally passes from the dens of the axis through the vertebra to the promontory of the sacrum. The center of gravity of the body is located just ventral to the sacral promontory ( Fig. 7-2 ). The vertebral column has different types of articulations: cartilaginous joints between the vertebral bodies, apophyseal joints between vertebral arches, unique articulations between the axis (C2) and atlas (C1), and skull-C1 articulation.


Vertebrae
Each vertebra consists of a cylindrically shaped body ventrally and an arch dorsally, and all encase the spinal cord and nerve roots. The outer shell of the vertebral body consists of a thin layer of relatively rigid compact cortical bone. This outer shell houses an inner core of soft and porous cancellous bone containing bone marrow. The structure of the cortical bone is aligned in vertical lamellae to resist compressive forces. The trabeculae of the cancellous bone are ordered like columns, and they resist a variety of loads. The rostral and caudal surfaces of the vertebral body are generally concave and are separated and bound together by the fibrocartilaginous discs. The dorsal arch is composed of the laminae, pedicles, spinous processes, and facet joints. Pedicles are stout bars of bone extending dorsolaterally from the rostral aspect of the vertebral body. The laminae extend dorsally, immediately from the pars interarticularis. They fuse in the midline to form the dorsal wall of the spinal canal. The laminae are oblong plates with a sloping surface. The spinous process arises from the junction of the laminae. The orientation of the spinous process depends on the region of the spine (cervical, thoracic, and lumbar). The cervical transverse processes arise from each side of the vertebral body near the junction of the pedicle and body. The thoracic and lumbar transverse processes arise from the junction of the pars interarticularis and pedicle. The transverse and spinous processes serve as attachments for muscles and ligaments. The articular processes arise from the pars interarticularis, interposed between the pedicles, laminae, and facet joints. Generally, superior articular processes project cranially with the articulating surface of facet on the dorsal surface. Typically, the inferior articular processes project caudally with the articular surface facing ventrally. A thin layer of hyaline cartilage lines the surface of each facet, which is a synovial joint, lined with synovium, and surrounded by a capsule. The following sections describe the characteristic features of cervical, thoracic, and lumbar vertebrae.
Cervical Vertebrae
These vertebrae are smaller in size compared with those in the thoracic and lumbar regions. They are cylindrically shaped and are wider in the transverse than anteroposterior (AP) diameters. The size gradually increases from C3 to C7. The pedicles are short and project dorsolaterally. They arise from the vertebral body midway between the rostral and caudal surfaces. The arch is composed of paired pedicles and articular facets, as well as the lamina and spinous processes. The laminae are narrow and overlap. The spinous processes are short and are usually bifid from C3 to C6. The transverse processes are unique. They contain the transverse foramen from C1 to C6, which transmits the vertebral artery. The anatomy of a typical cervical vertebra (C3-7) is shown in Figure 7-3 . The pars interarticularis in the cervical spine is termed the lateral masses . The superior and inferior facets extend from the lateral masses. The facets from C2-3 to C6-7 are oriented approximately 45 degrees with respect to the horizontal and are aligned with a coronal orientation to their surfaces. The first cervical vertebra (C1), or atlas, is ring shaped and supports the cranium. The atlas consists of a bony ring with stout lateral masses and anterior and posterior arches. It has large lateral masses containing the horizontally oriented facet surfaces. Rostral facets articulate with the occipital condyles of the skull, and the inferior facets articulate with the rostral facets of C2. The axis (C2) has a unique shape with a transitional morphology; it has a well-developed vertebral body with the odontoid process projecting rostrally. Its broad sloping superior facets extend laterally from the body.

Thoracic Vertebrae
These vertebrae are somewhat heart shaped and are intermediate in size between the lumbar and cervical vertebrae. The anatomy of a typical thoracic vertebra is shown in Figure 7-4A . It exhibits costal facets on each side at the junction of the body and pedicle and on transverse processes. These facets are unique ( Fig. 7-4B ). The costal facets are also seen on the transverse processes (except for T10-12). Vertebrae at the rostral and caudal regions have some transitional morphologic features—that is, T1 to T4 vertebrae have some cervical features, and T9 to T12 have some lumbar features. The surface area gradually increases from T1 to T12. The middle four vertebrae have almost equal lateral and AP dimensions. Lateral dimensions increase toward the cervical and lumbar extremes of the thoracic region. The spinous processes of the first, second, eleventh, and twelfth vertebrae are horizontal; the third, fourth, ninth, and tenth are oblique; and the fifth to eighth spinous processes overlap and are long and vertical. The size of transverse processes increases progressively from T1 to T12. The cervical features of T1 include the superior vertebral notch, and the lumbar features of T12 include the lateral direction of the inferior articular processes. The laminae are broad and sloping, and they overlap one another like shingles on a roof. The thoracic facets are oriented along the coronal plane. At the thoracolumbar junction, they assume a more oblique sagittal orientation.

Lumbar Vertebrae
Vertebral bodies in this region are the largest and typically increase in the diameter caudally. They are larger in the transverse width than their AP diameter; a concavity of the vertebral body gives rise to an hourglass profile and a kidney-shaped cross section. The bodies of L1-2 vertebrae are deeper dorsally. The L4-5 vertebrae are deeper ventrally, whereas the L3 vertebra is transitional. The laminae are relatively broad, wide, and minimally overlap. The interlaminar spaces are covered by the ligaments and by large oblong and horizontal spinous processes. Long, thin, slender horizontal transverse processes incline slightly rostrally in the lower two lumbar segments. The transverse process of L3 projects the farthest and that of L5 spreads ventrally. The fifth lumbar vertebra represents the transition from the lumbar to the sacral spine. It is substantially taller ventrally. This contributes to the lumbosacral angle. The thick and conical transverse process arises from the junction of the pars and the pedicle of L5. The anatomy of a typical lumbar vertebra is shown in Figure 7-5 .

Sacrum and Coccyx
The sacrum is formed by the fusion of the costal ligaments and the transverse processes. It is triangular in form, concave, and relatively smooth on its pelvic surface. It is convex and highly irregular dorsally. Five sacral bodies are demarcated by four transverse lines that end laterally in four pairs of ventral sacral foramina. The bilateral foramina are rounded laterally to indicate the courses of the emerging nerves. The coccyx may be a single bone fused from coccygeal elements, or the first segment may be separate from the other. These vertebrae are reduced in size, and they have no laminae, pedicles, or spinous processes.
Vertebral End Plates
These are formed by the rostral and caudal surfaces of the vertebral body. They are composed of concave surfaces of approximately 1.3-mm-thick cortical bone. The cartilaginous end plates are the superior and inferior thin planar surfaces of the intervertebral disc. They are the transition components between the fibrocartilaginous disc and the vertebral end plates. Each cartilaginous end plate is fused to the vertebral end plate by a calcium layer termed the lamina cribrosa , a sievelike surface that permits osmotic diffusion. Nutrients for the disc penetrate through the small pores at the lamina cribs.
Intervertebral Discs
The most rostral intervertebral disc space is located between the second and third cervical vertebrae and the most caudal disc is between the L5 and S1 vertebrae. Twenty-three discs span the vertebral column between C2 and S1. Discs demonstrate regional geometric variations that parallel morphologic differences in the vertebral bodies. The discs account for approximately one third to one fifth of the total height of the vertebral column. Four concentrically arranged components are often identified in the intervertebral discs: an outer alternating layer of collagen fibers that form the peripheral rim of the anulus fibrosus, a fibrocartilage component that forms a major portion of the anulus fibrosus, a transitional region between the central nucleus pulposus where the anulus and nucleus merge, and the nucleus pulposus. The core of the disc, termed the nucleus pulposus, is made of a soft, pulpy, highly elastic mucoprotein gel. The nucleus contains various mucopolysaccharides with relatively few collagen fibers and a high water content. The anulus fibers pass obliquely from the vertebral body above and below and are arranged in a helicoid manner. The anulus is composed of concentric layers of fibrous tissue. The orientation of the fibers within each layer is the same. The orientation of the fibers in adjacent layers differs by 30 degrees ( Fig. 7-6 ). The disc undergoes age-related changes. At birth, the disc has four distinct anatomic regions. However, the distinguishing features disappear as age transforms the disc into fibrocartilage and the number and size of the collagen fibers increases. With age, the macromolecular framework consists of collagen, proteoglycans, a noncollagenous matrix of proteins, glycol proteins, and small amounts of elastin. The elastic fibers are made of a central amorphous zone and a peripheral rim of dense microfibers. The arrangement of the fibers in the nucleus is irregular. The disc is approximately cylindrical with different ventral and dorsal heights. Typical cross sections of the disc resemble an ellipse in the cervical region, a rounded triangle in the thoracic region, and an ellipse in the lumbar region. Generally, midthoracic discs are mostly circular in cross section. In contrast, midcervical intervertebral discs are less circular. Like the vertebral body, cross-sectional areas of the disc increase from C2 to T1.

Ligaments
Ligaments are multilayered and are composed primarily of elastin and collagen. Ligaments connect adjacent vertebrae and may extend over several segments along the spinal column. Ligaments and joint capsules, while permitting normal spinal motion, restrict excessive motion.
Anterior Longitudinal Ligament
This ligament is continuous and spans the entire length of the vertebral column. It begins at the occiput as the anterior occipitoatlantal membrane and continues down to the sacrum covering one fourth to one third of the ventral circumference of the vertebral bodies and discs. It consists primarily of long-ranged collagen fibers aligned in interdigitizing layers. The deepest layer extends between the adjacent vertebrae, binding to the edges of the intervertebral discs. The middle layer binds the vertebral bodies and the discs over three levels, and the superficial fibers extend approximately four to five levels. The ligament is thickest over the concavity of the vertebral body blending into the periosteum.
Posterior Longitudinal Ligament
This ligament also traverses the entire length of the spinal column. It begins at C2 as the tectorial membrane and continues to the sacrum with fibers spreading out at the disc level and narrowing at the middle of the vertebral body. This ligament consists of several layers with the deep fibers extending only to adjacent vertebrae and the stronger superficial fibers spanning several levels. Whereas the ligament closely adheres to the disc anulus, it attaches only marginally to the vertebral body. This ligament is much thinner over the vertebral body and over the disc (by a factor of approximately one half) and is thickest in the thoracic region. Both the posterior and anterior longitudinal ligaments have a longitudinal (rostral to caudal) fiber orientation.
Ligamenta Flava
These are broad paired ligaments that connect spinal laminae. They arise from the ventral surface of the caudal lamina and attach to the dorsal border of the adjacent rostral lamina. They are discontinuous at midvertebral levels and in the midline. They extend laterally to the joint capsules and become confluent. These ligaments extend from C1-2 to L5-S1 levels. They have a high elastin content and are yellow. The ligamenta flava are the most elastic tissues in the human body. The capsular ligaments attach the adjacent vertebra to articular joints. The fibers are longer and slacker in the cervical region than in the thoracic and lumbar regions. The fibers are perpendicular to the plane of the articular surfaces.
Interspinous and Supraspinous Ligaments
These ligaments connect the adjacent spinous processes. They are composed predominantly of elastin. The interspinous ligaments attach from the base to the tip of each spinous process. They start at C2-3 and terminate at L5-S1. Both spinous ligaments are most prominent in the lumbar region. In contrast, the supraspinous ligament begins at the most dorsal aspect of the spinous process of C7 and continues into the lumbosacral region. The supraspinous ligament is primarily associated with the ligamentum nuchae of the neck contacting the spinous processes at their tips. It is the continuation of the ligamentum nuchae in the cervical spine. The ligament fibers end between the L3 and L5 levels. Figure 7-7A illustrates the ligamentous structures in the sagittal and axial planes.

Upper Cervical Spine Ligaments
Upper cervical ligaments span from the occiput to C2 (see Fig. 7-7B ). Beginning ventrally, the anterior longitudinal ligament is renamed as the anterior atlanto-occipital membrane from C1 to the occiput. The apical ligament attaches from the tip of the odontoid process of C2 to the basion of the occiput. The alar ligaments connect the rostrolateral aspect of the odontoid process and run obliquely to the occipital condyles. The cruciate ligament has ascending and descending bands and a strong transverse portion that courses dorsal to the odontoid process and attaches to tubercles on the medial aspects of the lateral masses of the atlas. The vertical cruciate ligament attaches from the occiput, just dorsal to the apical ligament, and intertwines with its transverse portion. The descending band attaches to the dorsocaudal aspect of the body of C2. The tectorial membrane attaches to the ventral one third of the basiocciput just dorsal to the vertical cruciate ligament. This ligament tapers caudally to become continuous with the posterior longitudinal ligament. Finally, the posterior atlanto-occipital membrane connects the rostral aspect of the dorsal arch of C1 to the occiput.
Muscles
The superficial muscles of the rostral thoracic region and dorsal neck originate from thoracic spinous processes and insert laterally on the scapula. The muscles are attached medially to the ligamentum nuchae, which is a fibrous intermuscular septum. The sternocleidomastoid muscles arise from the sternum and the clavicle, and they insert into the mastoid process of the occipital bone. In the lower thoracic and lumbar regions, several muscles make up the superficial layer. The most prominent muscle is the latissimus dorsi, which arises from the spinous processes of the lower thoracic vertebrae and extends as a sheet across to the ventral axilla. Both the intercostal muscles and serratus posterior muscles arise from the ribs in different directions. Muscles encircling the abdominal region include the external and internal obliques and the transversus abdominis. The rectus abdominis muscle is located in the ventral abdominal wall. Deeper muscles ventral to the vertebral column are less prominent than the dorsal muscles. In the cervical region, the longus coli muscle passes from the atlas to the transverse processes of C3 to C6. Deep lateral muscles include the anterior scalenus, the longus capitis, and the intertransverse muscles. They also attach to the transverse processes.
In the thoracic region, the longus coli muscle extends only a few segments. In the lower thoracic and upper lumbar region, however, the lateral muscle groups are prominent, especially the psoas, intertransverse, and quadratus lumborum muscles. The iliopsoas muscles originate from the lateral aspects of the vertebral bodies and extend to the femur. As in the rest of the spine, the intertransverse muscles extend between the transverse processes. The quadratus lumborum also originates from the transverse processes and runs obliquely to the lateral ileum. Beneath the trapezius muscle, the splenius capitis muscle arises from the lower ligamentum nuchae and the cervical and upper six thoracic transverse processes to attach to the occiput. The narrowest muscle, the splenius cervicis, originates only from the upper six thoracic spinous processes to insert on the posterior tubercles of C1 to C3. The adjacent deeper layer includes the semispinalis capitis and semispinalis cervicis muscles. The more medial semispinalis cervicis arises from the transverse and articular processes of the upper thoracic vertebrae inserting into the spinous process of the cervical spine. The lateral muscle originates from the transverse processes of C3 to C6 and inserts on the occipital bone. The deepest muscles of this group include the iliocostalis and longissimus cervicis, which arise from the upper thoracic ribs and transverse processes, respectively, to end on the transverse processes and facets of C4 to C7. Other deep muscles include the rectus capitis and capitis obliques, which serve as head extensors.
In the thoracic and lumbar regions, the erector spinae muscle group lies in the vertebrocostal groove directly under the thoracolumbar fascia. This muscle group begins as a tendon attached broadly to the dorsocaudal sacrum and iliac crest and extends the entire length of the spine. Its columns are composed of shorter fascicles. The lateral column represents the iliocostalis muscles, the intermediate column represents the longissimus muscles, and the middle column represents the semispinalis muscles.
The iliocostalis muscles arise from the iliac crest and insert on the angles of each of the ribs (iliocostalis lumborum and thoracis) as well as the cervical transverse processes. The longissimus represents the largest column. It arises from the transverse process at the lowest spinal levels and inserts into the transverse processes rostrally with the most rostral fibers inserting onto the mastoid process of the skull. The narrow spinalis muscle arises from the spinous processes of the sacrum and inserts into the higher spinous processes.
Deep to the erector spinae muscle lie the paravertebral or transverse spinal muscles. These muscles, including the semispinalis discussed previously, have their origins primarily from the vertebral transverse process and insert into the spinous process. The semispinalis group is continuous in the cervical and thoracic regions. The multifidus muscle is different in the cervical and lumbar areas, where the attachments are to the articular joint, but in the thoracic region the attachments are to the transverse processes. This muscle is thickest in the lumbar region.
Spinal Cord
The spinal cord and nerve roots traverse the spinal canal. The spinal cord is approximately 40 to 45 cm long in the adult and usually terminates at L1-2. The rostral cord at the level of the foramen magnum is continuous with the medulla oblongata. The dura mater, the pia mater, and the arachnoid are the three membranes that cover the spinal cord. The spinal cord is suspended in the spinal canal by dentate ligaments. These arise from the pia and are attached to the dura. Usually, the spinal cord terminates approximately at the caudal aspect of the L1 vertebral body. The cauda equina consists of the nerve roots, which have not exited through their neural foramina. Spinal nerves are composed of a dorsal sensory root and a ventral motor root. With the exception of the C1 and C2 contributions to the spinal accessory nerve, nerve roots leave the spinal canal via the neural foramina. Anatomically, the spinal cord is divided into five sections: 8 cervical, 12 thoracic, 5 lumbar, 5 sacral, and 1 coccygeal. Figure 7-8A shows a schematic of the spinal cord, indicating the relationship among spinal segments, nerves, and vertebral bodies.

The tracts within the spinal cord in the cervical and thoracic regions and nerve roots in the lumbar region are somatotopically oriented. The cortical spinal tracts are somatotopically arranged so that hand function is located more medially, whereas the foot function is located laterally. The spinothalamic tract is arranged so that hand sensation is located most medially and ventrally, and the sacral sensation is located most dorsally and laterally. The posterior columns are similarly arranged in a somatotopic manner. In the lumbar region, the nerve roots are arranged so that the lower sacral segments are located most medially and the exiting upper lumbar regions most laterally ( Fig. 7-8B ).
In a normal spine, spinal canal dimensions and hence the subarachnoid space are generous except in the midthoracic region ( Fig. 7-8C ). In the case of preexisting spinal stenosis, the factor of safety is reduced. This is important during a spinal instrumentation procedure that might impinge on the neural elements (e.g., sublaminar wire or hook placement). The lumbar spinal canal depth does not change significantly as one descends from the upper to the lower lumbar regions; however, its width increases (see Fig. 7-8C ). The lumbar and sacral spinal canal cross-sectional areas are also more generous than in other areas of the spine. They contain the cauda equina, which consists of peripheral nerves and is relatively resistant to traumatic insults. For both reasons, posttraumatic neural element injury in the lumbar region is less severe than that associated with comparable deformation in the other regions of the spinal column, particularly the midthoracic area. The respective shapes of the typical spinal canal in the cervical, thoracic, and lumbar regions are depicted in Figure 7-8D .
Fundamental Biomechanics
Biomechanics is defined as the application of the principles of engineering and computers to solve biologic problems. Clinical biomechanics of the spine refers to the understanding of the normal and the pathologic functions of the human vertebral column due to the application of mechanical insult. The insult could be in the form of traumatic dynamic forces, deformations, or slowly applied loads to the spine. Several terms are explained to facilitate a better understanding of clinical spinal biomechanics.
Scalar and Vector
A scalar is a quantity defined by its magnitude. It is directionally independent. Energy absorbed by the cervical spine due to the application of a load is an example of a scalar. In contrast, a vector possesses both magnitude and direction. Forces applied to the spine can be broken down into the components of vectors. To accomplish this, a reference system must be chosen. Force can be defined as an action that tends to change the state of the rest of the body to which it is applied. Force is a scalar. Force applied in a particular direction is often termed the force vector or load vector . In biomechanics, force and load are used synonymously. Because the spine is not rigid, the application of force results in deformations.
Cartesian Coordinate System
The right-handed Cartesian system of reference is commonly adopted in spine biomechanics. The system consists of three axes: x , y , and z . Rotational and translational movements can occur along and about these axes. Translational movements are considered positive if the movements occur along the positive direction of the axis; they are considered negative if the moments are in the negative direction. Similarly, a clockwise rotation around an axis looking from the origin of the coordinate system toward the positive direction of the axis is termed positive rotation, whereas the counterclockwise rotation is termed negative . Figure 7-9 illustrates the right-handed Cartesian coordinate system of reference with the z -axis oriented along the caudal to rostral direction, the x -axis along the dorsal to ventral direction, and the y -axis along the right-to-left direction. For the right-handed system, this results in a positive flexion moment (extension being negative), positive moment left-to-right lateral bending (right-to-left lateral bending is a negative moment), and positive twisting right axial rotation moment (left axial rotation is a negative moment). This reference system has been adopted by the American Standard for Testing Materials. Once the coordinate system of reference is chosen, the force vector can be divided into its components.

Deformation
Deformation can be translational or rotational. Translational deformation results in a change in the length of the body. Rotational deformation results in a change in the angle of the body to which the force is applied. Deformations result in strains.
Strain is defined as the change in unit length (linear) or change in unit angle (shear) in the body subjected to a force (vector). There are two types of strain: normal and shear. Normal strain is defined as the change in the length divided by the original length. Shear strain is defined as the change in the right angle (90 degrees). When a deformable body is subjected to a load vector, deformations occur, resulting in strains. The deformation along the direction of the force application is termed axial strain , whereas the deformation transverse to the direction of application of the force is often termed the transverse strain . The ratio of the lateral (transverse) to the longitudinal (axial) strain is termed Poisson’s ratio .
Kinetics and Kinematics
The study of the mechanics of the body in relation to the forces and the deformations is kinetics . In contrast, kinematics deals with the motion of the body (deformations) independent of the forces responsible for the deformations. Both the terms are applicable to the biomechanics of the spine.
Force Deformation Response
Because of the deformability characteristics of the spine, the application of an external force or a load vector results in deformations. Energy is frequently used to relate the force and the deformation; it represents the amount of work done by a force on a body. It is defined as the area under the force deformation curve. In contrast, stiffness is defined as the ratio of force to deformation. Because the force deformation characteristics of a spinal structure are not always linear ( Fig. 7-10 ), the most linear portion of the curve is often selected for obtaining the maximum stiffness of the structure. Analysis of the typical force deflection characteristics of the spinal structure (example of a functional unit) is given subsequently. Response is nonlinear—that is, force does not increase linearly with the deformation or vice versa. Within the principles of structural mechanics, this biomechanical load deflection response has been classified into the physiologic loading phase; the traumatic loading phase; and the failure, or the posttraumatic, loading phase. The stiffness response of the structure has been used to derive these biomechanical classifications. This system has been used to design a schema to evaluate the onset of spinal injury due to external load. This may help define the mechanism of spinal disorders.

In the physiologic loading phase, the spinal structure acts as an integral unit, and the stiffness increases gradually to a maximum value. During this phase, the structure obtains its highest stiffness; consequently, its resistance increases with the externally applied loads. This region represents the highest mechanical efficiency domain in the structural response. Trauma does not occur during this region of loading. With the increase in the application of load, yielding of the structures occurs. This is identified biomechanically by the onset of decreased stiffness for the first time during the loading process. Previous studies have demonstrated microfailures during this phase of loading. The end of this traumatic range is characterized by changes in the stiffness that correspond to the ultimate load-carrying capacity of the structure. After reaching its peak during the physiologic loading phase, the stiffness gradually decreases to zero at the end of the traumatic loading phase, indicating that the structure has reached its ultimate load-carrying capacity. In the subsequent phase (i.e., the posttraumatic loading phase), the structure responds with negative resistance—that is, an increase in the deformation results in a decrease of the load. Trauma has been identified on radiographs when the structure has been loaded to this level. Based on the simple fundamental force deformation response and using the stiffness as a mechanics-based criterion, studies have indicated that microtrauma may initiate the loss of a local component before the structure has reached its ultimate load-carrying capacity. In other words, even under subfailure loading, the structure may exhibit signs of weakness or microfailure.
Flexibility, Stiffness, and Range of Motion
Flexibility is defined as the inverse of stiffness (i.e., ratio of the deformation to an applied load). Flexibility and stiffness are inversely interchangeable in spinal biomechanics. Another quantity, the range of motion, is frequently used in spinal biomechanics. This refers to the deformation from one extreme to the other extreme under the physiologic range of translation or rotation of an intervertebral joint.
Coupling
Because of the three-dimensional nature of the spinal structure, motions are coupled. Coupling is defined as the capacity of the spine to move in translations or rotations independent of the principal motion. In other words, it represents obligatory movements of the spine (translations or rotations) that always accompany a primary motion. Both principal and coupled motions exist in the spine. Principal motion can be defined as the motion associated with the direction or the plane of application of the external force. Any out-of-phase motion is the coupled motion. For example, axial rotation of the upper cervical spine is usually coupled with lateral bending. Similarly, in the lower cervical spine, axial rotation and lateral bending of the vertebra in the opposite direction are usually coupled ( Fig. 7-11 ).

Bending Moment
The force vector may act on a lever arm to cause a bending moment. A diagram indicating the amount of bending moment at various sections of the structure is termed the bending moment diagram . Frequently, in spinal biomechanics, three-point bending ( Fig. 7-12A ) and four-point bending ( Fig. 7-12B ) are often used. In three-point bending, the force is applied at the middle of the length of a structure with the structure being supported at its two ends. This results in a triangular-shaped bending moment with the maximum moment under the point of load application. In four-point bending, the spinal structure is subjected to two equal loads placed at equal distances from the center, and the structure rests on two simple supports. This results in a trapezoidal-shaped bending moment diagram, in which the bending moment is constant between the points of load application. In this region, the sheer force is zero. A pure moment is sustained by the structure between the points of loading, and consequently the results obtained using a four-point bending technique can be applied for a pure bending moment situation.

Instantaneous Axis of Rotation
If the load is applied along the spinal long axis, it is called an axial or a longitudinal load . This load may result in structural buckling. The buckling load represents the highest load that the column can sustain before failure when the load is applied in the longitudinal manner. The instantaneous axis of rotation (IAR) defines characteristic movements during rotation of a vertebra. It is the point about which the vertebra rotates. The IAR is defined as the axis perpendicular to the plane of the motion of the body, and passing through a point within the confines of the body or outside the body that does not move is the IAR for that motion at that point in time. Table 7-1 includes additional details.
Description | Units |
---|---|
Displacement | mm |
Elastic modules | N/cm 2 |
Energy | N-m, J |
Force | N |
Moment | N-m |
Rotation | Degrees, rad |
Strain | Nondimensional |
Stress | N/cm 2, MPa |
Torque | N-m |
Stiffness | N/mm |
Flexibility | mm/N |
Clinical Biomechanics
Internal Deformation
The human spinal column resists external mechanical forces by undergoing internal deformations. The mechanical and structural changes depend on the type of force vector applied to the spine. The following terms are used routinely: flexion, extension, subluxation, rotation, and distraction ( Table 7-2 ). Flexion refers to a forward bending moment. Extension refers to a backward bending moment. Subluxation refers to an AP or posteroanterior shear. Rotation refers to an axial twist or torsion. Distraction refers to stretch or tension. Due to the anatomic characteristics of the vertebral column, the human spine is under the action of compressive force applied in an eccentric manner. Depending on the location of the center of gravity (see Fig. 7-2 ), this force generally induces a flexion moment. One of the principal actions of the vertebral body is to resist compressive forces. The compressive force resisted by the body gradually increases from the cervical to the lumbar levels. As described earlier, the width, depth, and height of the vertebral bodies increase from the rostral to caudal direction. This geometric phenomenon permits an efficient load-carrying capacity of the structure of the lumbar region compared with the cervical region. The exception is the height of the C6 vertebral body, which is less than C5 and C7; the height of the lower lumbar vertebral body is usually less than that of L2. Although the general shape of the vertebral body is cylindrical, the concave geometry of the dorsal aspect of the vertebral body (the surface facing the spinal canal) is significant in ventral spinal operations where screw purchase of the dorsal vertebral body cortex is critical. Misinterpretation of the lateral radiograph may lead to neural impingement by the screw. Figure 7-13 presents properties of the vertebrae from the cervical to the lumbar region. The compression strength of the vertebral body shown in Figure 7-13C indicates the general trend. Variations are due principally to the sample size, population, and age-dependent characteristics of specimens tested in the literature. An understanding of the tolerance levels under compression for the vertebral bodies is important in fracture fixation techniques.
Clinical | Bioengineering |
---|---|
Extension | Rearward bending |
Flexion | Forward bending |
Lateral flexion | Lateral bending |
Angulation | Rotation |
Rotation | Torque/twist |
Stretch/distraction | Tension |
Subluxation | Shear |

Facet Joints
The facet joints do not substantially support axial compressive loads unless the spine is in extension. Change in the orientation of the facet joints alters the mobility—and hence the load-carrying capacity—of the spinal column under different force vectors. For example, the primarily coronal orientation of the facet joints in the cervical spine, compared with an intermediate orientation in the thoracic region and a sagittal orientation in the lumbar region, account for the alterations in the magnitudes of the rotations of these regions. In particular, the facet joint orientation changes substantially from L1 (approximately 25 degrees) to L5-S1 (approximately 50 degrees). Figure 7-14 depicts the orientation of the facet joints in the cervical, thoracic, and lumbar regions. The general sagittal plane orientation of the facet joints in the lumbar region renders the lumbar spine unable to resist flexion or translational movements, whereas the ability to resist rotation is substantial ( Fig. 7-15 ). Relatively decreased incidents of subluxation found clinically can be attributed to a nearly coronal facet orientation at the L5-S1 level. It is well known that the subluxation is more common at L4-5 than at L5-S1 despite the relatively oblique orientation of the L5-S1 disc interspace. The ability of the cervical spine facet joints to resist flexion and extension, lateral bending, and rotation is relatively reduced because of the coronal plane orientation. Consequently, such movements are substantial in the cervical spinal region.


Spinal Cord
The spinal cord participates with the vertebral column in configuration changes due to alterations in body positioning. The susceptibility to injury varies with the specific abnormalities of the column. The physical properties of the spinal cord and related nerve roots, dentate ligaments, and pia and dura mater have been reported. The spinal cord is part of a continuous tract originating in the mesencephalon and extending to the point where the nerve roots exit. This structure participates in the physical alterations, with the predominant effects occurring at the local level of distraction. Similar to the biomechanical response of a functional spinal unit, distraction in the cadaver cord demonstrates a load displacement curve with two phases. Large initial displacements occur with small force levels, demonstrating the elastic flexibility of the cord. However, this initial flexibility is followed by stiffening in which additional stretch or distraction requires higher load levels.
In flexion, the spinal cord elongates within the spinal canal and decreases in the AP diameter. This induces increased axial tension in the axon cylinders of the white matter tracts and lesions of the vertebral canal that compromise the cross-sectional area, especially those processes ventral to the spinal cord call for the local and generalized increases in axial tension within the spinal cord. In extension, the spinal cord shortens and increases in the AP diameter with relative relaxation of the axon cylinders. The corresponding decreased cross-sectional area of the canal occurring from the dorsal bulging of the anulus, as well as the infolding of the ligamentum flavum and scaffolding of the lamina, may result in a “pincer-like” action on the cord. Studies indicate that irreversible spinal cord damage occurs when the compression exceeds approximately 30% of the initial cord diameter. Tensile forces applied to the spinal cord in the neutral position produce a relatively even load distribution across the structure, but if the cord undergoes bending, compressive forces increase on the concave side, causing increasing distractive forces on the convex side ( Fig. 7-16 ). Shear forces, in contrast to tensile forces, are maximal toward the center of the cord. By definition, shear forces act in a perpendicular plane to the tensile forces. Interaction of these force vectors applied to the various regions of the spinal cord during flexion indicates the potential for a complex pattern of injury. Increases in the shear stresses in the central region of the cord occur due to the “pincer-like” action, during a sudden forceful hyperextension.

Pedicle
The sagittal pedicle height increases gradually from the cervical to thoracolumbar region and decreases caudally in the lumbar spine. The transverse pedicle width decreases from the cervical to the midthoracic area and then increases caudally in the lumbar spine, favoring the placement of pedicle screws in the lumbar spine ( Fig. 7-17 ). Because of the generous dimension, a small variation in the pedicle height in the lumbar region is clinically insignificant ( Fig. 7-18 ). The decrease of the transverse pedicle angle from the cervical to the thoracolumbar region, and then a caudal increase in the lumbar spine, necessitates a wider angle of approach for the placement of pedicle screws in the lower lumbar spine ( Fig. 7-19 ). An appreciation of vertebral anatomy is important for pedicle screw placement in the sacral region. There is, however, usually a great margin of safety for screw placement in this region of the vertebral column.



Intervertebral Disc
Any load resisted by the vertebral body is transferred to the adjacent one (generally caudal) through the disc. Because of the inhomogeneity and anisotropy of the material properties of the body and disc, the mechanism of load transfer is complex. Differences in the age-related changes between the disc and vertebral body add to the complexity. Because of the relative flexibility of the disc compared with a very rigid cortical shell enclosing a relatively soft cancellous core, the intervertebral disc resists compression, tension, shear, bending, and torsion forces. Depending on the age and magnitude of the external load vector, any combination of these forces can result in complex three-dimensional deformations. Consequently, this component of the spine has received great attention by researchers. Similar to the vertebral body geometry, changes in the disc geometric properties account for the increases in the load to be resisted by the lumbar disc in contrast with the cervical intervertebral discs.
Unlike the vertebral body, discs cannot be tested in isolation—that is, the adjacent supports of the vertebra are necessary to determine its gross biomechanical properties. Routinely, functional segments (vertebra-disc-vertebra structure with or without the posterior complex) are used to determine the strength. Biomechanical testing has indicated that the first component to fail in a functional spinal unit is the end plate. In the lumbar spine, normal discs respond with higher load-carrying capacity and higher stiffness compared with degenerated discs. Studies have also demonstrated the movement of the nucleus material into the cancellous core of the vertebral body under discographic techniques. Initiation of trauma, identified biomechanically by the first decrease of stiffness within the specimen still able to resist further increases of load, occurs with the movement of the nucleus pulposus into the vertebral end plate, causing its rupture. Under symmetrical axial compressive loading, the disc bulges in the transverse plane. The compressive structural property of the disc does not contribute to the commonly observed disc herniation in the dorsal lateral direction; it depends primarily on the specific loading situations, which include compression, combined with other loading modes. Although the disc is never subjected to a direct uniform tension along its entire cross section, certain modes of loading induce tensile forces in different regions of the disc. For example, under physiologic conditions, the anulus of the disc is subjected to tensile forces. Under flexion, the dorsal aspect of the disc is subjected to tension; in extension, the ventral part of the disc experiences tension. In right lateral bending, tensile forces are resisted by the disc on the left side; in left lateral bending, tensile forces are resisted on the right side of the disc. From this point of view, the disc resists tensile forces locally, although the applied load vector may be in a different loading mode.
Depending on the spinal level, the disc resists a considerable amount of rotation or torsional forces. The average failure torque for a nondegenerated disc is 25% higher than it is for a degenerated disc. Similar to the case of an axial compressive load-deflection curve on a spinal unit, the torque versus angle curves are nonlinear. With increasing rotation, the torsional stiffness increases. Whereas the end-plate integrity alters in compression, under torsion the end plates generally remain intact. In experimental in vitro human cadaver studies, cracking sounds emanate secondary to injury to the anulus. This may have a clinical correlation in the fact that patients with low back pain often report that they have experienced a “pop” in the back.
Ligaments
Although ligaments possess three-dimensional geometry in terms of attachment and insertion, from a biomechanical perspective they are uniaxial structures—that is, they respond to direct tensile forces. Figure 7-20 illustrates the tensile load-carrying capacity of spinal ligaments. The effectiveness of a ligament depends on the morphology and the moment arm through which it acts. To appreciate the contribution of a ligament to the load-carrying capacity of the spine, one must consider the anatomic location as well as the strength of the ligament under tensile forces. A very strong ligament that functions through a relatively short lever arm may contribute less to the stability than a weaker ligament working through a longer lever arm ( Fig. 7-21 ). For example, although the posterior longitudinal ligament is relatively strong, it offers little resistance to flexion because of its ventral attachment. In other words, because the ligament is closer to the IAR than the interspinous ligament, it is less effective ( Fig. 7-22 ). This is similarly true for the ligamentum flavum. This ligament is deficient in the midline—that is, a longitudinal midline cleavage plane exists. This property facilitates surgical entrance to the epidural space. The ligamentum flavum is not lax except under hyperextension. This factor, along with its high elastin content, minimizes the likelihood of buckling during extension, which might result in dural sac compression. The dorsal location of the posterior longitudinal ligament relative to the IAR, combined with a shorter moment arm, renders it a weak resistor of flexion. Although the capsular ligaments have a shorter lever arm, particularly in the cervical spine, they play a large role in the maintenance of spinal stability. This stems from their increase of strength compared to their counterparts in the thoracic and lumbar regions.


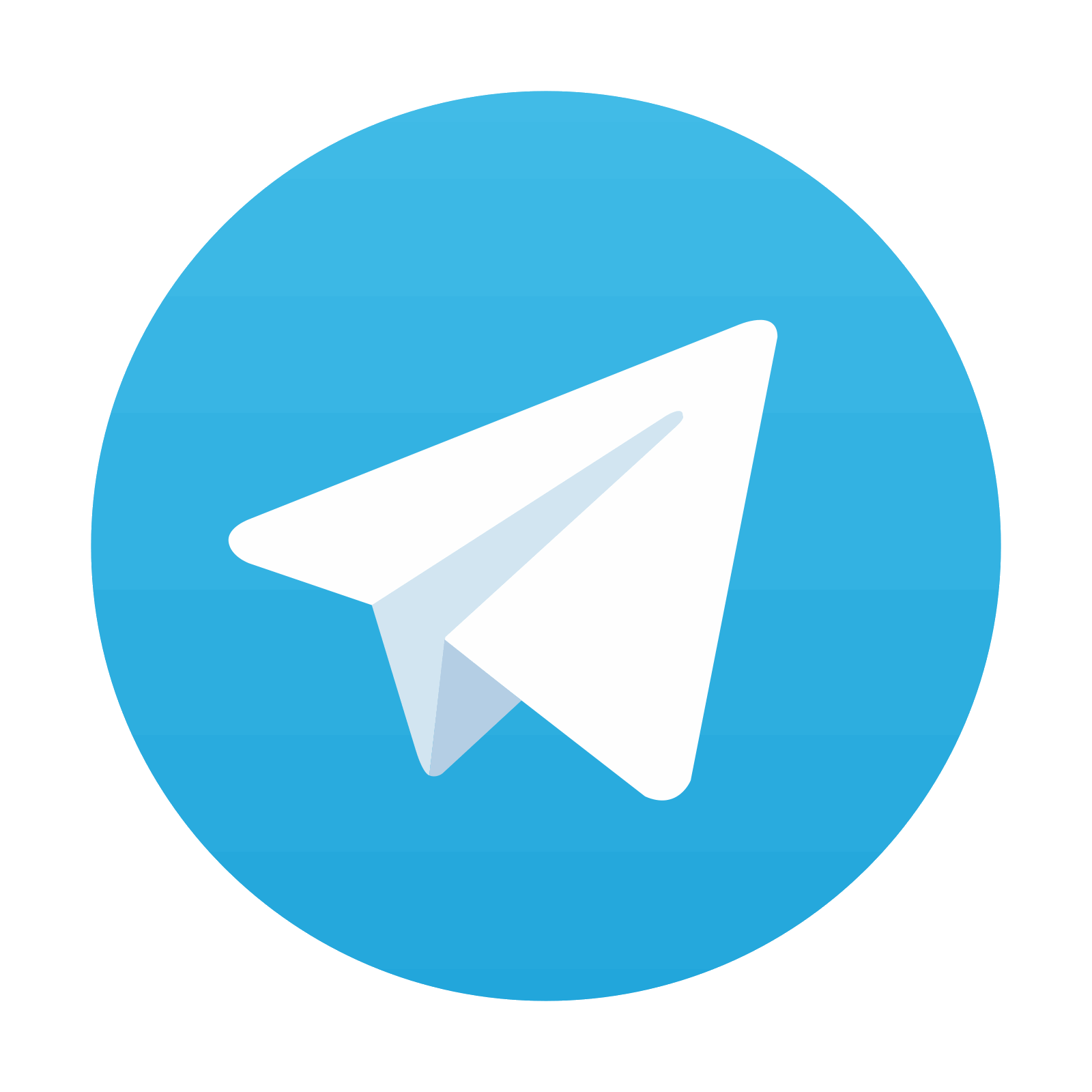
Stay updated, free articles. Join our Telegram channel
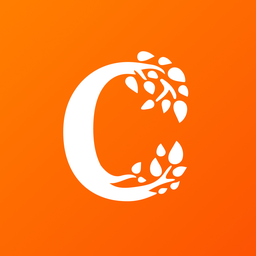
Full access? Get Clinical Tree
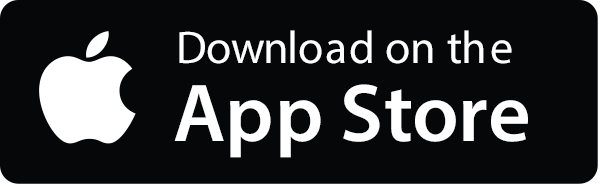
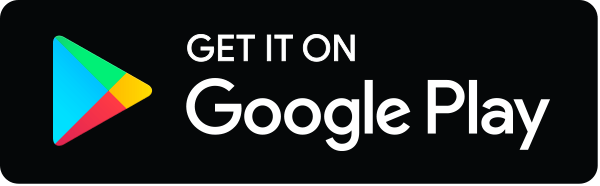
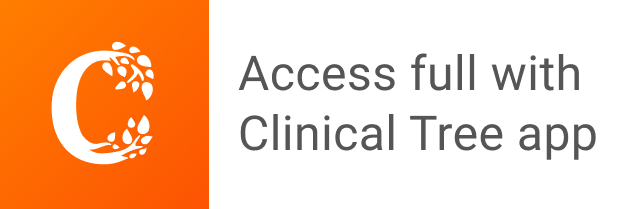