Fig. 10.1
Illustration of prefrontal cortical minicolumns, layers and loops. (a) Prefrontal cortical minicolumn highlighted in a human brain slice. (b) Laminar and columnar display of the major cell types in prefrontal cortex together with its connections to basal ganglia and thalamus. The six-layered cortex is showing on a Nissl background the pyramidal cells and the cortical interneurons. The cells are connected to the thalamus and basal ganglia, as shown on the lower panel. VTA is ventral tegmental area; N. accumbens is nucleus accumbens. (c) Pyramidal cell surrounded by interneurons illustrates the minicolumnar curtain o inhibition. AD is apical dendrite, BC is basket cell, BD is basal dendrite, CH is chandelier cell, DB is double bouquet cell. (d) Lateral inhibition function shows the neural activation (excitation and inhibition) with horizontal distance from a minicolumn’s center (Adapted from Opris and Casanova, 2014)
10.2 Prefrontal Cortical Minicolumns
Vernon Mountcastle described for the first time the electrophysiological basis of the cortical minicolumn and suggested it as an elemental unit of information processing (Mountcastle 1998, 1957, 1997; DeFelipe et al. 2012). According to this model of cortical organization, neurons and their connections form part of a vertical system which unites the cells of each minicolumn (Fig. 10.1a, b) into a coordinated functional unit (Mountcastle 1978, 1997). In this context, the smallest unit of cortical organization is the minicolumn, usually defined in Nissl stained sections by a narrow radial array of pyramidal neurons traversing laminae II-VI (Rakic 1988; Mountcastle 1997). Minicolumns are composed of vertical chains of excitatory neurons surrounded by inhibition (Fig. 10.1c, d) in cylindrical arrangement that constitutes the smallest module capable of information processing (Mountcastle 1957, 1998; Rakic 2008). The human neocortex is composed of a large number of minicolumns in parallel vertical arrays (Casanova et al. 2007). Minicolumns are the first step in a nested ensemble of nodes or “echelons” of increasing complexity (Opris and Casanova 2014). Other levels of modular organization include multiple minicolumns, macrocolumns, and large-scale networks of macrocolumns that are interconnected with the entire brain (Buxhoeveden and Casanova 2002). The somas of pyramidal cells are not randomly distributed in space; rather, they are organized into layers and different-sized columns or modules. Similarly, some dendritic and axonal ramifications that begin or end in these somas are wired in parallel groups of fibers (Mountcastle 1997, 2003). Minicolumns are often considered highly repetitive, even clone-like, units; however, they display considerable heterogeneity between areas and species, perhaps even within a given macrocolumn.
10.2.1 Columns and Minicolumns
Minicolumns are arranged within larger columns or macrocolumns (e.g., barrel somatosensory cortex of the rodent) bound together by short-range horizontal connections (Jones 2000; Zhang and Alloway 2006; Jones and Rakic 2010; DeFelipe et al. 2012). The different echelons are semi-independent of each other, a function of the limited number of information channels between them (Casanova 2005). According to Buxhoeveden and Casanova (2002), and in agreement with Favorov and coworkers (1987, 1990) and Mountcastle (1957, 1997), the estimated width of cortical macrocolumns is 350–600 μm. Hubel and Wiesel (1974) found that optimal orientation tuning changes systematically through 180° with an electrode advance of between 0.5 and 1.0 μm. The term “hypercolumn” refers to a complete rotation of columns (e.g. 0°, 10°, 20°,…,180°; Hubel and Wiesel 1974; Wiesel and Hubel 1974). Recently, Opris and collaborators (Opris et al. 2011, 2012b, c) suggested that interlaminar interaction in prefrontal cortical minicolumns take an active role in sensorimotor integration and the selection of behaviorally relevant targets. Sparse distributed representations of inputs flowing from visual cortex to the higher association areas in prefrontal cortex appear to be part of distributed networks, named “cognits” by Fuster (Fuster and Bressler 2012). However, the specific role of prefrontal macrocolumns in prospective coding and representation storage is yet to be demonstrated (Bastos et al. 2012).
A sparse distributed representation is one where items are encoded by activation of a small set of the available representing units. Sparse encoding does not reduce to a straight majority vote scheme. Anatomically, sparse encoding may be enforced by variability between minicolumns which themselves suggest differences in their internal architecture (Casanova 2008; Rinkus 2010). This variability among components of a minicolumn may contribute to the fault tolerance of larger networks such as macrocolumns. McCulloch (1959) has shown that when failure of individual components occur under a certain threshold (e.g., cell loss at the beginning stages of Alzheimer’s disease) redundant networks of unstable nets could be designed for greater reliability than redundant systems of stable nets of the same size.
10.2.2 Cortical Modules and Maps
Early studies by (Mountcastle et al. 1955; Mountcastle 1957, 1997) and Hubel and Wiesel (Hubel and Wiesel 1974; Wiesel and Hubel 1974) showed that neurons with similar response properties are grouped in vertical columns, about 0.5–1 mm in diameter, with each column oriented perpendicular to the surface of the cortex and spanning its thickness. Correspondingly, anatomical studies (using histological staining for the enzyme cytochrome oxidase) showed a modular organization in the primate visual cortex with periodically spaced patches about 350 μm apart (Horton and Adams 2005). In the primate prefrontal cortex the diameter of columns vary between 300 and 500 μm, but it does not differ significantly in size between brains with over three orders of magnitude difference in volume (Bugbee and Goldman-Rakic 1983). This common periodicity means that any block of cortex, approximately the size of a single hypercolumn, contains cells tuned to all values of every receptive field variable (Swindale et al. 2000). Hubel and Wiesel (Hubel 1982; Katz et al. 1989) applied the term “module” to this tissue block comprising multiple, overlapping hypercolumns. Mountcastle (1997) has used the term ‘module’ interchangeably with ‘column’.
The most salient feature of cortical organization is the presence of an orderly topographic map of visual space that is remapped sequentially as information flows from visual cortex to prefrontal cortex (Salinas 2004). Neighboring neurons tend to have receptive fields in similar positions in visual space, and these positions “change predictably” as a function of position on the cortex (Swindale et al. 2000). In addition to orientation, visual neurons vary in their preference for the direction of motion of an oriented bar or edge, in their preference for stimuli delivered to one eye or the other (ocular dominance), and in their preference for low versus high spatial frequencies in the visual image (Swindale 1998). All of these properties have been found to vary in an orderly way with position on the cortical surface, so that, typically, a complete set of values occurs at least once every mm or so.
10.3 Prefrontal Cortical Microcircuits
As proposed by Mountcastle, the primate neocortical circuitry has a modular architecture that subserves a multitude of sensory (visual, auditory, touch), motor, cognitive (attention, memory, decision) and emotional functions (Mountcastle 1957, 1997; Shepherd and Grillner 2010; Opris and Bruce 2005). According to this “modular” view of the prefrontal cortex, Goldman-Rakic (1996) provided evidence from experimental studies in nonhuman primates that the central executive decomposes into segregated information processing modules each with its own sensory, mnemonic, and motor control features.
Inter-laminar microcircuits, consisting of interconnected pyramidal neurons between the supra- and infra-granular layers, form a three stratum functional module that connects both worlds via sensory and motor circuits, in which infra-granular layers execute the associative computations elaborated in supra-granular layers (Buxhoeveden and Casanova 2002; Casanova et al. 2011; Thomson and Bannister 2003; Opris et al. 2011, 2012b, 2013). These microcircuits receive input from neurons in layer L4, which project to L2-L3, or through direct thalamic projections to the supra-granular layers in the higher-order cortical areas. Neurons in L2-L3 then project top-down to L5, where they target specific types of pyramidal cells and inhibitory interneurons. Some L5 neurons project back to L2-L3 neurons, forming an inter-laminar loop (Weiler et al. 2008) back to L4, targeting mostly interneurons (Thomson and Bannister 2003). The outputs from cortical microcircuits, cortico-striatal projections arise mostly from L5, whereas cortico-thalamic projections arise from L6.
Cortical microcircuits are connected by cortico-cortical connections into a macro-network that links areas within the same hemisphere, as well as across hemispheres (Van Essen et al. 1982). This super network subserves the ‘perception-to-action’ cycle – a group of processes that handle environmental stimuli and convert them into actions (Fuster and Bressler 2012; Romo et al. 2002). Microcircuits within the same hemisphere are interconnected (from low level sensory to high level associative processes) through horizontal connections in lamina 2/3, spanning over many cortical areas (Das and Gilbert 1995; Kritzer and Goldman-Rakic 1995; Fuster and Bressler 2012).
Inter-area connectivity of cortical microcircuits preserves spatial topography suggesting a column-to-column match from one area to another (Goldman-Rakic 1996). Additionally, the topography is preserved within minicolumns owing to the inter-laminar projections (Opris et al. 2013). Interhemispheric connectivity is formed by neural interconnections of lamina 3b (Jones 2000; Van Essen et al. 1982). Cortical microcircuits are also interconnected across the two hemispheres through cortico-cortical connections from lamina 3b (Jones 2000; Van Essen et al. 1982). This implies that the firing of a single callosal neuron might influence several cortical columns within the opposite hemisphere. However, microcircuits in each hemisphere are symmetrically interconnected (from low level sensory to high level associative processes) through horizontal connections in lamina 2/3 across many cortical areas (Das and Gilbert 1995; Kritzer and Goldman-Rakic 1995; Fuster and Bressler 2012). This imply a bottom-up remapping of a spatial topography with a column-to-column match from one area to another on the dorsal visual stream from V1 to prefrontal cortical area 46 (Goldman-Rakic 1996). In the same time each topography is preserved across layers through top-down inter-laminar projections within minicolumns (Opris et al. 2013).
Recent research conducted in nonhuman primates indicates that a variety of sensory, motor and executive functions emerge from the interactions between frontal, parietal, temporal and occipital cortical microcircuits (Atencio and Schreiner 2010; Buffalo et al. 2011; Opris et al. 2012b, c, 2013; Mahan and Georgopoulos 2013; Hirabayashi et al. 2013a, b; Takeuchi et al. 2011; Hansen et al. 2012). This suggests that cortical microcircuits perform elementary computations while cognitive functions are sub-served by a broader network comprising multiple cortical areas (Fuster and Bressler 2012). This implies that cortical microcircuit integrate, represent or select the relevant signals from a multitude of incoming inputs.
10.4 Integration, Representation and Selection in Cortical Microcircuits
The functional role of the cortical minicolumn is a continuing source of research and debate more than half a century after it was identified as a component of brain organization (Mountcastle 1957, 1997; Mountcastle et al. 1955). Nevertheless, the cognitive ability of prefrontal cortical mechanism is hypothesized to emerge from the “laminar-columnar” architecture of the prefrontal cortical minicolumns, that are interconnected (Fig. 10.2) with basal ganglia and thalamus in recurrent loops (Bugbee and Goldman-Rakic 1983; Goldman-Rakic 1996; Opris et al. 2011, 2012d, 2013). Such columnar recurrent ‘microcircuit’ may be regarded as the ‘basic functional unit’ of the cognitive function. Its cognitive relevance emerges from the ‘computational’ ability of the inter-laminar microcircuit to: (a) integrate incoming signals of the input layers, (b) store information through feedback connections in reverberatory loops, and (c) to compare input signals to a threshold criterion, triggering an output response i.e. the ability to make a decision.


Fig. 10.2
Inter-laminar microcircuits for integration, representation and selection. (a) Prefrontal cortical integration of cognitive, oculomotor, motor and limbic structures in four loops. (b) Illustration of bottom-up and top-down processing within cortical microcircuits
10.4.1 Integration
The integrative role of cortical minicolumns as a module (Leise 1990) stems from connecting the horizontal and vertical components of the cortex within the same columnar space. The supragranular layers L2/3 which are the major source of corticocortical projections also receive sensory information, while the infra-granular layer L5 is the output to subcortical structures involved in behavior (Miller and Cohen 2001). Thus, interlaminar connections form microcircuits that bind sensory-related signals with behavior/movement related outputs (Opris et al. 2011). This sensorimotor integration (Fig. 10.2a) was demonstrated by Opris et al. (2011, 2013) by means of interlaminar correlated firing between supragranular layers that carry perceptual/visual spatial information and the infra-granular layers that carry action related information. Such transformations of neural signals may likely reduce the output degrees of freedom within the cortical minicolumn by selecting only the relevant signals for action/behavior. This integrative process that occurs in canonical microcircuits binds/segregates parallel streams of minicolumnar processing within the ‘executive cognit’ network (Fuster and Bressler 2012; Miller and Cohen 2001).
Prefrontal microcircuits are in a unique and privileged position at the top of sensory-to-motor hierarchy network because they coordinate a multitude of stimuli, perceptions, biases and actions related to such functions as attention, decision making, and working memory. As such, prefrontal microcircuits integrate and synthetize signals over a broad spectrum of perceptual stimuli and various modalities (Wilson et al. 1993). This integration is performed in supra-granular layers, whereas the output of the infra-granular layers provides selection-related signals, which are sent back to the infra-granular layers and the other areas comprising the network. As a matter of fact, signals can reverberate within inter-laminar loops. Thus, microcircuits in entorhinal cortex and hippocampal formation employ such reverberating signals (Takeuchi et al. 2011) to integrate relevant information over time (Fuster 2001). For example, microcircuits of the temporal cortex use such synchrony to maintain items in long term memory (Takeuchi et al. 2011; Hirabayashi et al. 2013a), while the microcircuits in the prefrontal cortex perform elementary computations for the executive control of behavior (Opris et al. 2012b, c);
10.4.2 Representation
The prefrontal cortex is crucial for the representation of stimulus properties (spatial, object features) in working memory and the hippocampus is essential for the long term memory (Funahashi et al. 1989; Miller and Cohen 2001; Takeuchi et al. 2011; Naya and Suzuki 2011). Prefrontal cortical “modules” (minicolumns) are composed of neurons with “memory fields” (Goldman-Rakic 1996), with isodirectional tuning for minicolumnar cells (Rao et al. 1999). Prefrontal neurons exhibit persistent “delay period” activity with enhanced firing rate in animals performing delay response tasks, being regarded as a neural “signature” of working memory (Funahashi et al. 1989). Such persistent firing of prefrontal cortical neurons is hypothesized to emerge from functional interactions between cells in different cortical layers, wired together in reverberatory loops (inter-laminar and/or thalamo-cortical) (Alexander et al. 1986). As a matter of fact, signals can reverberate within inter-laminar loops (Weiler et al. 2008). Thus, cortical microcircuits for long term memory in entorhinal cortex employ such reverberating signals (Takeuchi et al. 2011) to represent relevant information over time (Fuster 2001). Takeuchi et al. (2011) demonstrated a “reversal” of interlaminar signal between sensory and memory processing in monkey temporal cortex. Thus, during the sensory “cue” epoch, the canonical microcircuit signals flowed “feed-forward” from granular to supragranular layers and from supragranular to infragranular layers, while during the “memory” delay epoch, however, the signal flow reversed to the “feed-back” direction: from infragranular to supragranular layers. Such reversal of signal flow highlights a neat dissociation of the sensory and mnemonic processing in the temporal cortex that differentially recruits its laminar circuits.
10.4.3 Selection and Decision Making
The available evidence suggests that the prefrontal cortical minicolumn might be the first stage bottleneck in the cortical-striatal-palidal-thalamo-cortical loop (Alexander et al. 1986). It is obvious that layers 2/3 and 4 cells integrate a lot of inputs from virtually all of the brain, while the number of outputs from layer 5/6 pyramidal cells to subcortical structures participating in behavior is much less. Also, a key role in selection is played by the GABAergic interneurons of minicolumns (Raghanti et al. 2010) that shape the tuning for preferred direction/location by means of lateral inhibition. The minicolumnar role in plasticity is associated not only with the tuning (Fig. 10.3) of synaptic activity states but also with optimal selection among alternate subnetworks of microcircuits developing within a given context. Such parallel subnetworks may process complementary submodalities within a defined receptive/memory field; alternatively they may provide overlapping response characteristics to a common input. Competition among networks allows for circuit optimization (selection), in particular by means of learning.


Fig. 10.3
Simultaneous recordings of cortical minicolumns. Minicolumnar interlaminar processing of target selection during the match phase of the task. Peri-event and cross-correlation (post- vs. pre-match) histograms depict the functional role in integration/selection of prefrontal cortical layers and minicolumns
We hypothesize that minicolumnar diversity provides the substrate for this competition and the basis for adapting learned behavior to context. During development, neurogenetic programs interact with epigenetic factors to regulate formation of cortical microcircuit templates (Rakic 1988; Jones 2000; Jones and Rakic 2010; Kaas 2012), which are then shaped and pruned by differential patterns of sensory activity. Thus, increased minicolumnar diversity may give rise to greater potential for combinatorial activity of microcircuits within overlapping networks, resulting in enhanced learning and behavioral flexibility (Casanova 2008). Cortical minicolumns may therefore play a crucial role in behavioral selection that is in fact the substrate of executive function (e.g., attention, decision making).
Is cortical minicolumn a decision module? A decision circuit is defined as a closed neural network that measures the probable value of a signal element and makes an output signal based on the value of the input signal and a predetermined criterion or threshold (Ratclif et al. 2003; Opris and Casanova 2014). Minicolumns in PFC are interconnected to each other through horizontal “long range” projections in layer 2/3 (Kritzer and Goldman-Rakic 1995; Rao et al. 1999) and interlaminar mini-loops (Weiler et al. Takeuchi et al. 2011). The loop is then closed (“reverberatory loops”) through projections to the subcortical basal ganglia nuclei and thalamus (Alexander et al. 1986; Swadlow et al. 2002). Such “reverberatory loops” may be regarded as the “basic functional unit” of cognitive/executive mechanism because they: (i) combine incoming signals of the different input layers (Casanova et al. 2007); (ii) store mnemonic information through feedback connections in “persistent” spiking activity (Wang 2012); (iii) compare input signals to a threshold criterion triggering an output response (selection), which constitutes the ability to make a decision (Ratcliff et al. 2003). Thus, a cortical minicolumn with integrative, selective and threshold abilities can play the role of a decision module.
10.5 Correlated vs. Causal Relationships to Executive Control
Several approaches based on microcircuits have been implemented. These advances have been possible owing to of the development of new multi-electrode arrays (MEA) fitted for recordings from neural elements of cortical columns (Hampson et al. 2004; Moxon et al. 2004). Thus, MEAs with linear or bi-linear geometry have been successfully employed for simultaneous recordings from supra- and infragranular cortical laminae in adjacent minicolumns, resulting in unprecedented insights into the function of cortical microcircuits (Mo et al. 2011, Opris et al. 2011, 2012b, c, 2013).
10.5.1 Inter-laminar Interactions and Emergence of Executive Control
The relevance of minicolumnar activity to executive function has been investigated with different approaches under several conditions (Hirata and Sawaguchi 2008; Opris et al. 2011, 2012b, c, 2013; Hampson et al. 2012). Our recent results in nonhuman primates show for the first time interlaminar processing in PFC (Fig. 10.3) during target selection (Opris et al. 2011, 2012b, c) and sensorimotor integration (Opris et al. 2011). An example of this interlaminar interaction during target selection (Opris et al. 2012b, c) in delay match to sample (DMS) task is shown in Fig. 10.3 for two cell pairs with rasters and perievent histograms (PEHs) bracketing the temporal interval of image presentation (Match Phase onset) and completion of the target selection Match Response (0–2 s). The cell pairs were recorded on appropriate sets of adjacent pads (minicolumns 1&2) in the conformal multiple electrode array (MEA) shown in the illustration (Opris et al. 2011, 2012b, c) of both interlaminar cell pairs in L2/3 and L5 (Fig. 10.3 center). Neurons in both layers showed significant increases in mean firing in supra- and infragranular layers as a function of Match presentation (Post Match: 0 to +2 s) and during subsequent movements associated with target selection. Demonstration of precise functional connections between individual cells within each minicolumn was provided by cross correlation histograms (CCHs; Opris et al. 2011, 2012b, c); constructed for individual L2/3 and L5 cell pairs recorded on vertically positioned pads of the MEA. Normalized CCHs for both minicolumn cell pairs and between minicolumns are shown in Fig. 10.3 for cell firing in the displayed PEHs: (i) prior to (black) Match phase onset (2 s to 0, Pre) or (ii) after (green) Match phase onset (0 to +2 s, Post) for the same cell pairs. Both CCHs show significantly correlated firing (Opris et al. 2012b).
10.5.2 Causal Relations Involving Inter-laminar Microcircuits
The unique properties of conformal MEAs (Fig. 10.4a; Moxon et al. 2004) together with prior microstimulation work (Opris et al. 2001, 2005; Hampson et al. 2004) has provided a basis for showing functional relationships to executive function in prefrontal cortex of nonhuman primates. The conformal multi-electrode array (MEAs) also provide the basis for applying a system specific model to control firing of cells via application of electrical stimulation (Opris et al. 2012c; Hampson et al. 2012) to the same loci in which columnar firing has been detected and analyzed with respect to DMS task performance (Opris et al. 2012c; Hampson et al. 2012). This same model was implemented to test whether it could facilitate performance on trials that show a distinctive difference in correct performance (Hampson et al. 2012) as a function of the prior instructions as to type of response to make in the Match phase (i.e. Object vs. Spatial trials). Figure 10.4 shows the integration of a multi-input multi-output (MIMO) nonlinear math model to assess the patterns of firing in L2/3 and L5 cells recorded in the columnar manner with the MEA shown with adjacent vertical pads (Opris et al. 2011, 2012b; Hampson et al. 2012). Figure 10.4b reflects the type of input and output firing patterns recorded and analyzed by the MIMO model and also illustrates how the output pattern of L5 cell firing is duplicated via a multichannel stimulator that is capable of delivering predetermined patterns of pulses to the same L5 pads to mimic firing on correct trials. The advantage of the MIMO model is that the online recording provides the means to detect when the inappropriate L2/3 firing pattern occurs which triggers the delivery of the appropriate L5 stimulation pattern providing the means to override errors and enhance performance (Hampson et al. 2012). Stimulation consisted of 1.0 ms bipolar pulses (20–50 μA) delivered to L5 recording locations following presentation of the Match phase screen and prior to the completion of the Match Response.


Fig. 10.4
Manipulation of executive control in PFC microcircuits. (a) Prefrontal striatal neuronal circuits. (b) Simultaneous recording and MIMO model microstimulation. (c) Cumulative microstimulation effect neuronal circuits. (d) Population mean performance as a function of the number of images in the task. (e) Distribution of stimulation induced tuning vectors
The results of microstimulation delivery are shown in Fig. 10.4c, d, in which the effects on performance are compared to trials in which stimulation was not delivered, respective of trial type. Figure 10.4c compares the change in % correct performance as a function of processing time (reaction time + movement time) on stimulation (Stim) trials with respect to the no stimulation (No stim) case. Figure 10.4d showed for the first time the increase in correct performance on trials as a function of the number of distracter images in the Match phase. The results indicate that MIMO derived stimulation induces enhanced cognitive processing (Opris et al. 2012c, 2013; Hampson et al. 2012) required to retrieve the “rule for successful selection” of the appropriate item. The distribution of microstimulation induced spatial bias (Fig. 10.4e) will be shown in Fig. 10.5 of the next section.


Fig. 10.5
Prefrontal cortical microcircuits and the perception to action cycle. (a) Perception to action cycle. (b) Behavioral DMS task. (c) Task performance as a function of the number of images and delays in the DMS task. (d) Neural activity in prefrontal cortical layers L2/3, L5 and caudate during perception and selection. (e) Spatial tuning during the perception and selection phases of the task. (f) Distribution of MIMO stimulation induced tuning. (g) Distribution of neural tuning in prefrontal cortical layers L2/3, L5 and caudate during perception. (h) Distribution of neural tuning in prefrontal cortical layers L2/3, L5 and caudate during executive control/selection. (i) Population cross-correlation between prefrontal layers L5 and caudate nucleus during match vs. pre-match phase. Compiled figure from Opris et al. 2013
10.6 Prefrontal Microcircuits Bind Perception to Executive Control
A broad range of brain functions, from perceptual to executive actions encode, represent, monitor and select information that is either spatial- and/or object-specific for effective behavioral performance (Quintana and Fuster 1999; Goldman-Rakic 1996; Posner and Snyder 1975; Shallice and Burgess 1996; Botvinick et al. 1999; Selemon and Goldman-Rakic 1988; Opris and Bruce 2005). Such constellations of brain abilities use large scale neural circuits consisting of thalamo-cortical loops and cortical microcircuits with functional roles in the integration, representation and selection of information (Fuster and Bressler 2012; Alexander et al. 1986; Opris et al. 2011). Cortical microcircuits topographically connected by cortico-cortical and subcortical connections into a super network subserves the ‘perception-to-action’ cycle – an ensemble of processes that sense/perceive the environmental stimuli and convert them into actions (Fuster and Bressler 2012; Romo et al. 2002).
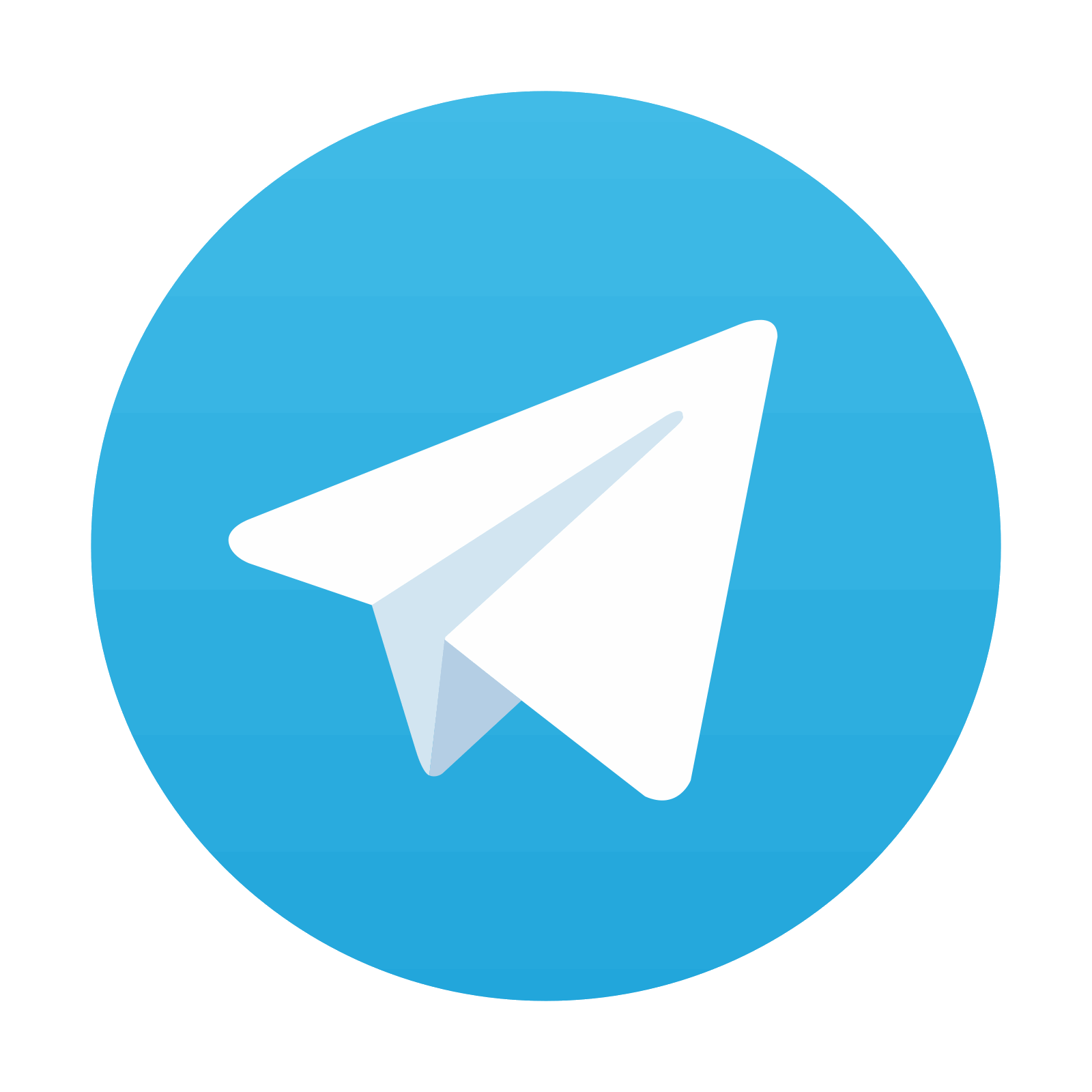
Stay updated, free articles. Join our Telegram channel
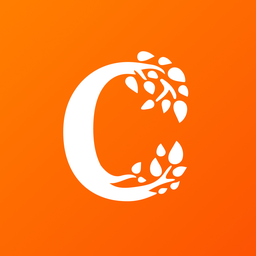
Full access? Get Clinical Tree
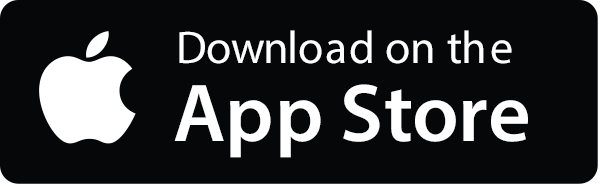
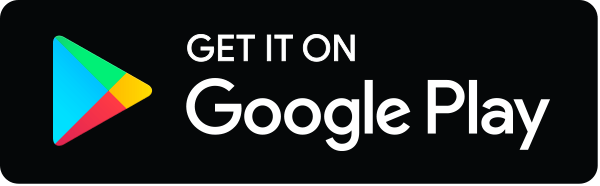