(1) Inadequate or threats to airway
(2) Impaired ventilation or respirations
(3) Significant external or suspected internal hemorrhage
(4) Abnormal neurologic status
a. Glasgow Coma Score (GCS) < 14
b. Seizure
c. Neurologic deficit
(5) Penetrating trauma to head, neck, torso, or extremities proximal to knee/elbow.
(6) Amputations or near-amputations proximal to fingers/toes
Despite the widespread use of GCS for assessment of the multisystem trauma patient with traumatic brain injury , there is significant concern, particularly among EMS physicians, regarding comparative value, accuracy and reproducibility of this and other assessment classification scores for head injury [29–38]. Both PHTLS and ITLS include determination of Glasgow Coma Score during the primary and secondary trauma survey, though one utilizes a rough assessment of the level of responsiveness on an Alert-Verbal-Pain-Unresponsive scale (AVPU scale) at the point of initial contact as a rapid gauge of neurologic status.
Numerous authors have noted the limitations of the GCS and the p-GCS in particular. The GCS is difficult for EMS personnel to consistently remember and accurately apply without ready references and frequent experience with its use—particularly in the highly stressful context of a multisystem trauma patient in the prehospital setting [32, 34, 39]. The GCS was not intended to be used as a single score but rather the expanded components are more valuable [29, 40]. The GCS is less reliable in the mid-range of scores from 9–12 [29]. The predictive value of the GCS for mortality is less sensitive in elderly patients at traditional threshold values [41]. Hypotension, hypoxia, and language barriers all impact the GCS [29]. The Brain Trauma Foundation (BTF) Guidelines recommendation for the use of the GCS is a weak recommendation based on low quality, Class III evidence [42]. The BTF EMS guidelines also call for the correction of hypoxia and hypotension prior to obtaining GCS, which is rarely the case with the initial p-GCS [42]. Although the guidelines suggest that the prehospital GCS is a reliable indicator of severity of TBI, evidence suggests p-GCS lacks prognostic value although observed trends in GCS are more predictive [35]. In the multiply injured trauma patient, the interpretation of GCS is even more complex.
Notably once acquired, many guidelines stratify GCS into ordinal categories of injury severity—mild brain injury with a GCS 13–15, moderate brain injury with a GCS 9–12, and severe brain injury with a GCS 3–8. The AVPU scale produces a similar stratification by injury severity (Table 5.2)
Table 5.2
AVPU scale and corresponding GCS range and injury severity
AVPU responsiveness category | Likely associated GCS | Classification of severity of injury |
---|---|---|
Alert | 13 (E4/V4+/M5+) | Mild GCS 13–15 |
Verbal | 12 (E3/V4+/M5+) | Moderate GCS 9–12 |
Painful | 6 (E2/V2+/M2–4) | Severe GCS 3–8 |
Unresponsive | 3 (E1/V1/M1) |
It has been suggested that simpler and more reproducible measures may perform equally and with greater consistency than the GCS. Some suggest that the 6-point motor score alone provides adequate performance to replace the 15-point full GCS [29–31, 43]. The KISS principle and reproducibility under stress would favor utilization of even simpler scales such as the Simplified Motor Scale (also called the TROLL exam with a built-in scoring mnemonic for EMS—Test Responsiveness: Obeys—Localizes—or Less) [43, 44].
Simplified motor score (SMS) | TROLL exam |
---|---|
2—Follows commands | 2—Obeys commands |
1—Localizes painful stimuli | 1—Localizes painful stimuli |
0—Withdraws to pain or less | 0—Less than localizes painful stimuli |
The SMS has been validated in two independent studies in both the ED and the prehospital setting [43–45]. It provides the same relevant information as GCS. It was statistically derived and shows better inter-rater reliability than the GCS [32, 33, 45].
While GCS likely will still play a role in the trauma bay and ICU, strong consideration for less complex and more reproducible scoring systems such as SMS or AVPU should be considered for prehospital providers and the unique prehospital phase of care, at least in the early phases of patient assessment and stabilization.
Despite being a pillar of ATLS guidelines for hospital-based treatment, airway management is one of the most complicated and controversial in the prehospital arena [27, 46–48]. Numerous studies have failed to demonstrate any survival benefit in moderately to severely injured trauma patients undergoing prehospital intubation [49–55]. Several authors have found an increased mortality, though more recently there have been studies that showed an outcome benefit [51, 56]. There are several reasons why prehospital intubation may not be in the best interest of the patient, even in the setting of severe TBI. First, while seasoned medics may tell “war stories” about intubating an entrapped patient upside down in a dark, muddy ditch, none would argue that these are ideal conditions for a high-risk procedure, and even the best prehospital conditions are nowhere near those of the trauma bay or operating room [46–48, 57–59]. The ability to obtain and then maintain proficiency in endotracheal intubation is also a challenge for many EMS systems. The studies that have been able to demonstrate outcome improvements in the setting of prehospital rapid sequence of the patient used small, highly practiced cohorts of prehospital providers that perform intubations frequently [51, 60]. This does not describe the vast majority of EMS systems. Though it is possible that advances in high fidelity simulation can compensate to some degree, regular access to the operating room may be both necessary and increasingly uncommon [61–64]. It is likely that paramedics need to intubate 12–15 times per year to maintain proficiency [65]. This number can be difficult to achieve in systems with either low call volumes or a high number of advanced life support providers. Using blinded pulse oximetry and ETC02 data, it has been shown that EMS providers frequently underestimate both the difficulty and time involved during an individual intubation, which is particularly relevant as maximizing physiologic parameters to prevent secondary injury is a current mainstay of brain injury treatment [66, 67]. Single episode of hypoxia has been associated with a worse outcome, and the circumstances involved in a severely brain-injured patient often do not allow for ideal intubation conditions [66, 68–72]. A relatively new concept of delayed sequence intubation, which involves procedural sedation with ketamine followed by aggressive preoxygenation prior to paralysis and endotracheal tube placement, has been studied among altered mental status patients in medical setting, but currently there is no published study on its use in trauma [73–77].
To some degree, the older prehospital rapid sequence intubation (RSI) literature needs to be interpreted in the context of changes in technology. Video assisted laryngoscopy has replaced direct laryngoscopy in many systems, and research has shown that paramedics can obtain intubation proficiency quicker with these devices [78–81]. A number of supraglottic rescue devices have replaced surgical cricothyroidotomy as backup for failed intubation and this too may influence success rates [82–86]. Concerns about decreased cerebral perfusion in the setting of supraglottic airways have been raised in animal models [87]. However, a recent radiologic study showed no decrease in carotid flow in humans [88]. Unrecognized esophageal intubation or tube dislodgment during transport can be mitigated by routine use of waveform capnography [89, 90].
Even if intubation is successful and uncomplicated, there are significant risks that still must be managed. Inadvertent prehospital hyperventilation is well-documented, even in experienced providers [69, 91, 92]. This can lead to cerebral vasoconstriction, decreased cerebral perfusion, and ultimately increased injury to at risk areas of brain [71, 93]. This again can be potentially mitigated by strict use of continuous and EtCO2 monitoring, ventilation timing devices, and transport ventilators [89, 92]. While end-tidal CO2 is an unreliable index of PaCO2 in the hemodynamically unstable, multisystem trauma patient, it is the only available means to guide ventilations [94–96]. Maintaining end-tidal in the 35–45 mm Hg range is recommended for the intubated patient though no recommendations are available for the nonintubated patient supported by supraglottic airway or bag valve mask ventilation [89, 97].
The introduction of positive pressure ventilation, particularly over-ventilation, can decrease effective perfusion by decreasing thoracic venous blood return. This has been associated with poorer outcomes even in the setting of normal CO2 levels, a concept widely referred to in the prehospital setting as “death by hyperventilation [98].” We will discuss the concept of therapeutic hyperventilation in the setting of suspected herniation syndrome later in this chapter.
The management of volume status in the trauma patient has evolved greatly in the last 30 years. While we have largely moved past the days of 2 large bore IVs run wide open in every trauma patient regardless of presentation, balancing resuscitation goals in the brain-injured polytrauma patient remains quite challenging [99]. The benefits of controlled resuscitation are most well established for penetrating injury with noncontrollable source of blood loss [99–103]. The role of controlled resuscitation in blunt multisystem trauma is still evolving and questions remain regarding which patients, how low, and how long [102, 104–106]. In blunt trauma with brain injury the optimal strategy, and detailed thresholds remain unclear [51, 70–72, 99, 103]. Strategies appropriate for isolated brain injury may not directly apply to brain-injured patient with uncontrolled bleeding from an extracranial injury. Similarly, controlled resuscitation strategies reasonably considered for torso injury, may be harmful in the patient with associated severe TBI [101, 102, 104, 107, 108]. In fact, many of the controlled resuscitation studies excluded head injured patients. Studies including patients with intracranial injury show increased mortality in head injured patients in whom fluids were withheld in the setting of hypotension, and a single episode of early hypotension has been associated with up to a 150 % increase in mortality [70, 72, 107, 109].
Studies which included head injured patients show a trend toward benefit with IV fluid administration and correction of hypotension [107]. However, the appropriate resuscitation endpoint remains ill defined. Interestingly, the 2007 BTF guidelines use a SBP of <90 mm Hg as the definition of hypotension [42]. Some authors and evidence suggests increased mortality at threshold of SBP <110 mm Hg [105, 109–111]. While it may be that mean arterial pressure is a more appropriate measure to guide resuscitation and to achieve and maintain an adequate cerebral perfusion pressure in the face of presumed elevations in intracranial pressure, the studies showing adverse outcome reported SBP rather than MAP. For these reasons most guidelines present their recommendations in terms of SBP rather than MAP.
In the prehospital environment, there are significant limitations that impact end points for resuscitations. Blood pressure is most frequently obtained by a noninvasive oscillometric automated cuff, and accuracy of devices in the setting of hypotension may be variable [112, 113]. The manually auscultated blood pressures that are often used upon arrival in the trauma bay can be limited in feasibility due to noise during air or ground transport. Palpated blood pressures and pulse oximetry wave form systolic blood pressure may be variably utilized when circumstances preclude auscultation. The precision and reliability of such measures are unclear and the evidence on thresholds for targeted resuscitation does not address the method of monitoring [114].
Currently, utilizing the proposed thresholds by alternative monitoring methods is also not validated. As continuous capnographic monitoring has become common, there have been suggestions to use EtCO2 as a marker of perfusion [94–96]. While this appears to have utility in medical cardiac arrest patients, in the multisystem trauma patient, dynamic changes in both ventilatory and hemodynamic variables are likely to impact the observed values and the correlation to PaCO2 is less reliable and less predictable [59–61]. The validity of this measure in nonintubated patients and patients with supraglottic airway devices is unknown.
Possible clinical endpoints such as palpable radial pulses and mental status rather than numerical endpoints may be best suited for the prehospital setting, though distinguishing alternations in mental status due to inadequate perfusion as opposed to primary TBI (or even simply alcohol intoxication) is challenging.
Strategies for diagnosing and managing herniation syndromes in the prehospital setting is an area warranting particular discussion, as the recommendation of limited hyperventilation of the patient with suspected impending herniation—“targeted or therapeutic hyperventilation”—by BTF, ITLS, and PHTLS influences not only the individual patient but also may unintentionally impact on the care of the population of brain-injured patients as a whole.
The BTF prehospital guidelines suggest that clinical signs such as dilated and unreactive pupils, asymmetric pupils, abnormal motor response with extensor posturing or unresponsiveness, or deteriorating GCS from a starting score of 9 be used to identify herniation, though they stipulate that ventilation, oxygenation and hemodynamics be normalized before considering targeted hyperventilation and that it be discontinued when signs are no longer present [42]. However, the success of a targeted strategy presupposes the reliability of the clinical signs. That is not clearly established to be true in the prehospital setting. This is concerning since herniation protocols rely on accurately identifying the population that may benefit from hyperventilation and distinguish it from the non-herniating population known to be harmed by hyperventilation.
It is reported that as much as 17 % of the population has anisicoria and was noted to be pronounced in 4 % of the studied population [115]. A retrospective analysis found that pupil asymmetry had a positive predictive value for an intracranial lesion of only 30 % and even with asymmetry greater than 3 mm the positive predictive value was only 43 % [116]. The authors note “a single measurement of pupil asymmetry is neither a sensitive nor specific finding in either identifying or localizing an intracranial lesion.” [116] A fixed pupil is defined in the guidelines as less than 1 mm of response to bright light. The accuracy of estimates in a field environment is unknown and, because metabolic and cardiovascular abnormalities including hypotension, hypoxia, and hypothermia all may be associated with dilated pupils and decreased reactivity, guidelines state that pupils should be assessed after the patient is resuscitated and stabilized [117]. The BTF prehospital guidelines on pupillary exam note that the relation between prehospital pupillary findings and outcome have not been evaluated and recommendations are based on studies from in-hospital studies where pupillary findings have prognostic value predicting mortality, and studies have found that while pupillary function may be an indicator of brain injury after trauma, it is not a specific indicator of severity [116, 117].
When hyperventilation is utilized in the hospital setting the guidelines recommend using it in conjunction with ICP monitoring to guide therapy. The prehospital environment substitutes clinical measures out of necessity but this almost certainly decreases the accuracy of appropriate patient identification. The use of a targeted strategy on a population level also supposes that the intervention has a high likelihood of benefit in the appropriate population compared to the magnitude and frequency of harm with which it will be applied to the non-herniating population. This is a function of both the size of the populations of interest and the size of the harm/benefit in the respective populations. The incidence of cerebral herniation has been estimated to occur in 40 % of cases of severe TBI although the frequency with which it occurs within the prehospital phase of care is likely even lower [51, 72, 118]. For these reasons, the majority of cases of severe TBI that prehospital providers encounter are likely to involve TBI without cerebral herniation.
Hyperventilation reduces ICP at the expense of cerebral blood flow (CBF) and cerebral perfusion pressure (CPP). The effects on CBF are compounded in the multisystem trauma patient with TBI whose injuries place them at greater risk for superimposed hypotension [51, 72, 103]. The beneficial effects of hyperventilation are at best short-lived and predicated on delaying herniation to allow more definitive interventions. Although weak recommendations from BTF advocate limited short-term hyperventilation in narrowly selected patients facing imminent risk of herniation, no outcome studies establish its effects [69, 93, 97]. In light of the adverse effects associated with hyperventilation when applied to non-herniating patients, it is reasonable to consider more narrowly defined selection criteria as well as pursue alternative treatments with less potential for adverse impact. These may include simple measures such as elevation of the head of the bed to 30°, keeping the head midline and avoiding compression of venous drainage.
Hyperosmotic agents are variably utilized in the prehospital setting, more commonly by air medical providers and critical care interfacilty transport services than ground-based emergency response services. The majority of evidence comes from hospital-based studies including the use of hypertonic saline (HTS) as a hyperosmotic agent in the management of elevated ICP, which will be addressed elsewhere in this text. Since high concentration HTS requires central venous access for administration, it is not suited for prehospital application. However, lower concentrations can be administered through peripheral venous access, as can 8.4 % sodium bicarbonate, which is readily available and familiar to all tiers of prehospital providers and been reported to reduce ICP in TBI patients for 6 h [51].
With all strategies for management of possible herniation, the best possible care for the individual patient in ideal circumstances must be balanced against the likelihood that the variables to inform such decision-making will be available in the prehospital phase of care and the potential downsides of misapplication of the strategy to a patient that is not suffering from the condition.
Transport modality and destination choice are the final major treatment decisions. The need to balance the logistics of patient movement with time sensitive medical intervention is one of the greatest challenges in trauma care, especially in the prehospital phase. Just as traumatologists must decide if a patient can be stabilized for arterial embolization in interventional radiology or needs to go directly to the operating room, prehospital providers must make multiple decisions that balance care priorities with speed to hospital. As we routinely tell our providers, all decisions, including logistical ones, are medical decisions. The thinking on when to transport has evolved considerably since the concept of the Golden Hour was first introduced. What started as “diesel fuel and cold steel” had swung considerably in the other direction of two large bore IVs prior to transport by the time a seminal 1996 study found that victims of penetrating trauma had a higher survival rate if they arrived in the ED via private vehicle as opposed to by EMS [119–122]. The seemingly simple decision of when to place an IV access needs to take into account what will be given through the line and what effect will it have on the patient’s overall outcome, balanced against delaying transport for the procedure and the likelihood of successful placement (and possibility of provider injury) in the back of a speeding ambulance.
The “right answer” continues to evolve with our knowledge treatment options. The data on tranexamic acid infusions from the CRASH studies has added more value to an IV, and the development of a successful blood replacement product could markedly rebalance the equation [123–127]. Regardless, because much of our current strategies for management of acute traumatic brain injuries involves maximizing physiology to prevent further damage, a thoughtful, multidisciplinary approach with active EMS engagement is most likely to result in the best logistical medical decisions.
When considering mode of transport, three main variables come into play. The first, and likely most important in the vast number of severely injured patients, is time to definitive care. In the civilian setting, this usually represents a choice between ground ambulance and HEMS transport. Ground assets are much more prevalent. In 2011, air medical transports represented approximately 2 % of all ambulance transports in the US, with only 1/3 being scene runs [23]. Availability of air assets is a function of geography, but also of weather conditions, appropriate landing zones, and other flight safety conditions. While one might assume that medical helicopters, with average cruising speeds of 135–150 mph and largely straight, traffic-free flight paths would be the quickest way to the hospital, the scene logistics play are often much more complicated [60, 128–135]. In most geographic areas, air assets are not routinely dispatched as part of EMS response, and once requested, there are still inevitable delays for weather/route checks and spin-up time [136, 137]. Additional delays can occur if a landing zone cannot be safely established immediately proximal to the scene, and thus requiring ground transportation to another location to meet the aircraft. At the aircraft, beyond the necessary pauses for patient report and physical transfer, noise, lighting and physical space limitations can necessitate patient assessments and invasive procedures, especially endotracheal intubation, be completed prior to on-loading. In situations where patient access is delayed due to entrapment or environmental barriers and the aircraft can be on the ground prior to packaging, many of these potential delays no longer apply. A conceivable gross rule of thumb could be, with regards to speed of arrival, patients benefit from air ambulance transport if the trauma center is greater than 30 min away [23]. In general, research seems to show improved outcomes with HEMS transport in patients with a moderate injury severity score (ISS) . Patients with severe ISS are unlikely to survive in any mode of transport, and HEMS resources seem to be unnecessary, from the physiologic standpoint, in patients with low ISS [60, 128–133, 137, 138].
Aside for the potential for more rapid transport to the trauma center, HEMS assets can also be utilized to bring providers with advanced training and protocols. Depending on the nature of the injuries, a time delay in transfer to definitive care may actually increase the chances of a good outcome [60, 131, 133]. Most would argue that a delay in transport to RSI a burn patient with the potential for significant airway involvement would be justified, while delaying transport in penetrating thoracoabdominal trauma is rarely in the patient’s interest [108, 122, 139]. Many of our brain-injured polytrauma patients fall somewhere in between these bookend cases. As referenced earlier, rapid sequence intubation in cases of suspected TBI may be associated with survival benefit. With a small number of providers with advanced training and regular practice, it is plausible that flight programs may better be able to manage the risks associated with RSI than EMS systems with less regular exposure to critical trauma [60, 128, 133].
The third variable—the impact on resource availability and population health—is less within the scope of this book, other than to acknowledge that the complexity of some logistical decisions. For example, the choice to drive a patient by ground 60 miles to the closest trauma center must be balanced by the risk to the population of taking the town’s only ambulance out of service for 2 h [60, 140–142].
The choice of destination hospital is a product of national recommendations, state legislative requirements, local trauma system guidance, hospital and EMS resources, and the specific injuries and/or mechanism of the individual patient. The National Expert Panel on Field Triage provides a decision algorithm through the CDC Trauma Triage Guidelines . It is recommended that patients with substantial alterations in level of consciousness or vital signs or with anatomically severe injuries such as penetrating thoracic trauma or two or more long bone fractures are transported to the highest level of trauma center available in the specific local trauma system [143]. Patients with a high-risk mechanism of injury but that does not meet the vital sign or anatomic criteria higher in the algorithm should still be transported to a trauma center but may not need the highest level of trauma care [143]. Local factors can have significant impact on destination decision as well. In 2005, 84 % of all US residents have access to a Level I or II trauma center within 60 min, though over one quarter would require HEMS to achieve timely access [140]. Depending on the region of the country, there maybe multiple Level I trauma centers in a five-mile radius or one in the entire state [135, 141, 144, 145]. EMS resources, both in terms of certification level and transport resources, can also vary considerably. Situations can arise where direct transport to a local non-trauma hospital is indicated for stabilization of a critical airway or tube thoracostomy prior to transfer to a trauma center for definitive care. These decisions can be complicated but may also have significant impact on individual patient outcome. A regional approach to trauma care involving perspective and expertise from all aspects of the care continuum is most likely to lead to good decision-making at the side of the patient [146, 147].
The last phase of EMS care for the trauma patient (other than the post-event analysis, debriefing, and feedback for which we strongly advocate), is the patient report and handoff . There are several points worth emphasizing during this phase. Despite numerous studies documenting the challenges on in-hospital sign-outs, there has been less formal study of EMS-ED handoffs. Research has generally found that between 25 and 50 % of EMS report is not acquired by the ED team [148–152]. This can be addressed in several ways. It is likely that simple awareness may lead to better communications. The nature of the problem lends itself to practice in cross-disciplinary simulation settings. It is possible that communications could be improved if EMS report follows a scripted format though the research has found mixed results [152]. One such tool is the “DeMIST” format—Demographics, Mechanism, Injuries identified, Signs (objective data like vital signs, EtCO2), Treatments and response to interventions [152]. Special attention should be paid to accurate and reliable information—vitals should never be “stable” and normal vitals must be entirely normal. Particular attention should be paid to trends over transport. A blood pressure on arrival of 110/60 may not be particularly alarming, but if it was 180/90 on-scene and 140/70 just prior to the hospital, the value is much less reassuring. Similar diligence should be paid to neurological findings particularly level of consciousness and pupillary exam. Related, if a patient has undergone prehospital rapid sequence intubation , communication of a field neurological exam, time and type of paralytic, time of last sedative, and evidence of patient motion since pharmacological paralysis can all help guide the care of the severely brain-injured patient.
The development of an integrated, coordinated, and effective trauma system that delivers high quality, well-researched, and evidence-based care across the continuum of providers is now a goal more than 50 years in the making. The 1966 National Academy of Sciences white paper, the 1996 “EMS Agenda for the Future” and the 2006 Institute of Medicine “EMS at the Crossroads” report all have broadcast calls to action to continue to improve the care of trauma patients in this country [1, 146, 147]. Continued interdisciplinary collaboration, whether at the side of a specific patient, in after-action discussions, regional steering meetings or books such as this will remain foundational in advancing our care of these complex and challenging patients.
References
1.
Division of Medical Sicences. Committee on trauma and committee on shock, national academy of sciences. Accidental Death and Disability: The Neglected Disease of Modern Society; 1966.
2.
3.
Zachariah BS, Pepe PE. The development of emergency medical dispatch in the USA: a historical perspective. Eur J Emerg Med. 1995;2(3):109–12.CrossRefPubMed
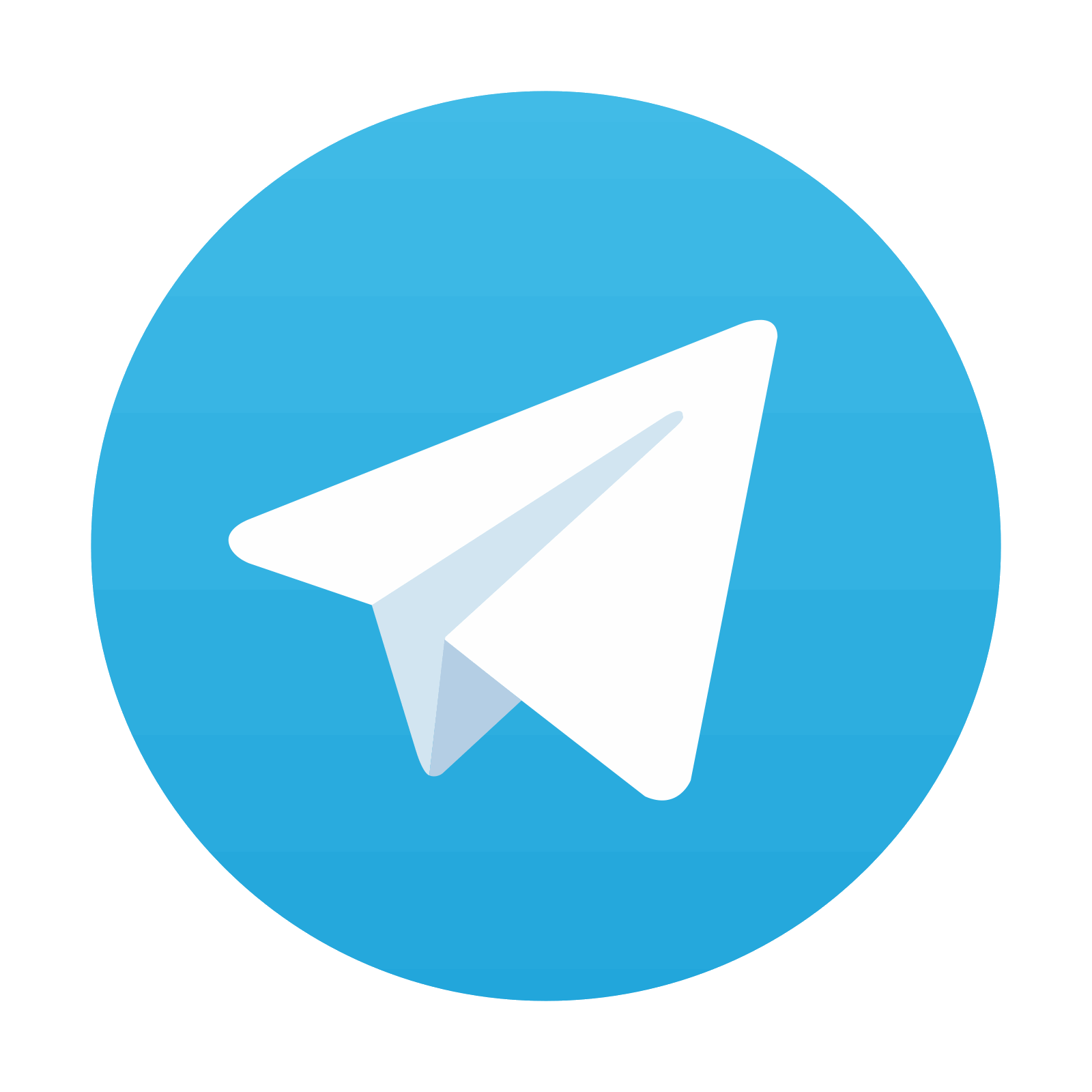
Stay updated, free articles. Join our Telegram channel
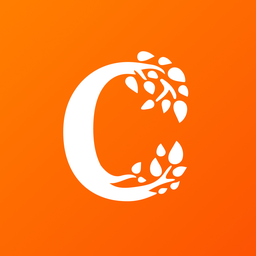
Full access? Get Clinical Tree
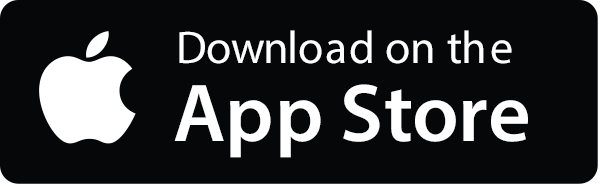
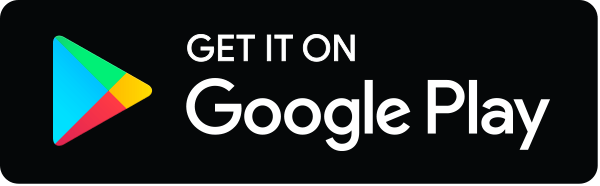