Chapter 3 Electrical properties of tissues
INTRODUCTION
The concept of bioelectrics is certainly not new, and the links between bioelectrics and electrotherapy have been proposed on numerous occasions (e.g. Charman 1990, 2002). It is not surprising that these suggestions have attracted a degree of scepticism, but looking objectively at the research literature from several fields (physiology, bioengineering, biophysics, cell biology), the integration of existing knowledge into a new discipline (or at least a subdiscipline) is possibly the most logical way forward.
Not only does bioelectricity and bioelectric tissue activity have an influence on the practice of electrotherapy (Chapter 19 ‘Electrical stimulation for enhanced wound healing’ is an example), but there is a strong case for its potential to influence manual and exercise therapies and a range of ‘alternative’ and ‘complementary’ therapies, which, although, beyond the remit of this chapter, and indeed this whole text, might be of significance in the future development of therapy.
THE ELECTRIC CELL
The transmembrane potential, readily accepted as a normal phenomenon, is an example of bioelectric activity on a small scale, although there is evidence that bioelectric activity is present and important at smaller (subcellular) scales. This section will largely avoid bioelectrics at a subcellular level, not because it lacks importance or evidence but rather because the larger scale is more understandable as an introduction and is more easily appreciated in a therapeutic context. Those with an interest in subcellular bioelectrics are encouraged to consider material by Charman (1990, 2002) and Oschman (2005).
The electrical potential across the cell membrane will average several 10s of millivolts (typical values cited for nerve membrane for example would be in the order of 70mV). This potential is actively generated rather than an accidental event and is dependent on the continued pump and ion transport system of the cell membrane for its maintenance. Ion concentrations are deliberately made unequal (against the tendency for diffusion gradients to generate ionic and thus a charge balance) and there are many different ionic transfer mechanisms across any cell membrane – from the now well-known and well-understood sodium-potassium pump (Na/K) through to the less well-known, but equally important, calcium ion gated channels (Charman 2002).
A transmembrane voltage (of, say, 70mV) is difficult to imagine. A cell membrane thickness of a few nanometres is more difficult, so by scaling both measurements up by the same factor, it is possible to grasp the magnitude of this energy system on a more ‘human’ scale. If we were to make the cell membrane a metre thick, the equivalent voltage across that membrane would be in the order of 7 million volts. Given that this is the equivalent potential in every living cell in the body, it constitutes an impressive energy system, and one that will clearly ‘cost’ the body to maintain. It is important to stress that this is the equivalent rather than the actual voltage across the cell membrane – what it would be if the cell membrane was a metre thick – which clearly it is not. One could then multiply up this membrane potential by the number of cells in the body and come up with some very impressive large numbers and an impressive amount of electrical activity.
The level of ‘excitement’ in the cell membrane is strongly linked to these physiological changes at a cellular level, and thus linked with many aspects of medical and therapeutic intervention. Cells can be forced, or encouraged, to increase activity levels – using drugs, heat or other energy sources such as ultrasound or laser light. Confining the argument here to the use of energy in the therapeutic environment, the absorption of energy at cell membrane level is partly, if not totally, responsible for the cellular upregulation seen with modalities such as ultrasound and laser, and almost certainly pulsed shortwave. Evidence that these modalities are able to exert their influence primarily at a cell membrane level (Cleary 1987, Karu 1987, Mortimer & Dyson 1988) supports a transduction mechanism by which the delivery of energy to the tissues is able to generate physiological (and thus therapeutic) effects (see Chapter 1).
TISSUE BATTERIES
THE BONE BATTERY
The bone battery is a well-documented physiological reality. In the 1950s, it was found that bone demonstrated a piezoelectric potential when subjected to physical (bending) stress (Fukada & Yasuda 1957); this work has since been extended by many researchers (reviewed in Black 1987, Guzelsu & Walsh 1993, Walsh & Guzelsu 1993). The demonstration of piezoelectric phenomena in bone is illustrated in Figure 3.1.
This work was extended in the 1960s by the demonstration of streaming potentials (Pollack et al 1984) and, more importantly than both of these (in the context of this chapter), in the late 1960s and 1970s by the steady potential (or battery potential) (reviewed in Black 1987). The streaming potentials are generated in bone when it is physically stressed and are a result of charged particles (ions) moving within the fluid-filled Haversian systems; more recently, they have also been demonstrated in cartilage (Legare et al 2002). Both the piezoelectric and streaming potentials are therefore stress related – they occur when bone (in this instance) is physically stressed. The steady potentials are measurable even when the bone is not physically stressed – they are there all of the time. It is these latter potentials that are derived directly from the ‘bone battery’.
The magnitude of the electrical potentials generated by the bone battery will vary between individuals (in keeping with almost all physiological phenomena) but if one considers the overall electrical pattern of a long bone, the femur for example, the gross electrical charge pattern will be similar between individuals, even if the actual values are variable (Rubinacci et al 1984).
The bone battery charge is physiologically relevant in that bone electricity varies when the bone is actively growing (Black 1987), when subjected to abnormal mechanical stress (e.g. Becker & Selden 1985) and when subjected to a pathological state (Borgens et al 1989, Marino 1988). Not only does the ‘charge’ generated by the battery vary under normal and pathological conditions, there is also a relationship between battery output and tissue injury/repair. When bone is subjected to injury, there is a physical discontinuity in the battery structure (the fracture) and a local electrical change will result. This local ‘current of injury’ has been demonstrated in many musculoskeletal tissues; bone in this instance is just an example.
It is argued that this local electrical current – or disturbance from the normal – is an essential driver for the process of fracture repair, and that without it, repair of the damaged bone will be inhibited or might indeed be absent (Black 1987). Becker (1974a, b) reports the experimental evidence with regard to fracture healing and denervation. It was noted that there was retardation of fracture healing in peripherally denervated extremities and that, when the nerve transection gap is bridged by the supporting (Schwann) cells (but not yet by the neurons themselves), the fracture healing rate was returned to normal. This is considered to link the local bone injury potentials with the direct current (DC) control theory (see the section ‘Direct current (DC) control theory’, below).
It is further argued that for individuals in whom this electrical activity is for some reason absent or reduced, a delayed or non-union is an inevitable consequence. It would be presumptuous to suggest that the only reason for non- or delayed union relates entirely to this ‘flat battery’ function but there is substantial experimental and clinical evidence to support the link between the phenomena (Black 1987).
There are many further relevant issues related to the bone battery, its existence and behaviour, but the primary issue in the context of this chapter is that the bone battery – internally generated electrical potentials coming from the bone tissue rather than conducted there by some other tissue – has an integral and important influence on bone physiology in health and disease. Extensive reviews have been written in this area and some accessible work is available (Black 1987, Evans et al 2001, Singh & Katz 1989).
There are therefore some obvious correlates between the electrical activity in bone, the process of fracture healing and the role of various aspects of the rehabilitation process. Given that the level of electrical activity in bone changes as a result of physical stress, and that it has been shown that bone bioelectric activity is an essential element of a ‘normal’ repair process, there is a strong argument that active exercise, manual therapy and various forms of electrotherapy are able to influence fracture healing by their capacity to directly or indirectly influence the bioelectric environment. That is not to say that the only influence of exercise or manual therapy is as a ‘battery’ stimulant, but just to make the point that in addition to the established value of these interventions in terms of local blood flow, increased muscular and general limb activity, mobility and strength, part of their mechanism of action is very likely to relate to enhanced bioelectric function. This would certainly be the case in relation to skin and wound healing (see the next section) and is directly related to the promotion of this activity by means of electrical stimulation (see Chapter 19). The enhancement of bone healing has been demonstrated by several different types of electrotherapy intervention, including ultrasound (e.g. Gebauer et al 2005, Malizos et al 2006, Warden et al 2006), laser therapy (da Silva & Camilli 2006, Garavello-Freitas et al 2003, Luger et al 1998), magnetic fields (Bassett 1989, 1993, Ryaby 1998, Trock 2000) and electrical stimulation (Anglen 2003, Ciombor & Aaron 2005, Park & Silva 2004) and although these might not constitute a major aspect of the context of this text, they are important and emergent issues in current electro-therapy practice.
THE SKIN BATTERY
The skin battery itself exists across the living layers of the epidermis and is oriented such that the external surface of the skin is always electrically negative in relation to the internal environment of the body (Barker et al 1982). The role of this battery function is similar in many respects to that of the bone battery already described. It is important as part of the maintenance of healthy skin function, and appears to be of critical importance in terms of the response of the skin to injury and the subsequent repair phases.
The mammalian skin potential work of Barker et al (1982) and Jaffe & Vanable (1984), using a skin injury model in guinea-pigs, has demonstrated that there is a current flow (movement of positive ions) out from a skin lesion due to an altered skin battery function. The skin potential at the wound site is zero because the battery has been short circuited, although, a few millimetres away, a normal transcutaneous potential exists. There is a lateral voltage gradient in the superficial tissues, the mean strength of which was 140mV/mm in guinea-pig skin. The lateral gradient was not present if the wound was allowed to dry. The model predicts a current flow from the intact skin battery into the deep wound, with a return path through the wound and to the tissue layer between the dead and living layers of the epidermis. The wound current is illustrated in Figure 3.2. Close to the wound, the outer surface of the living layer would be electrically positive with respect to the outer surface of the same layer far from the wound.
The strength of the lateral voltage gradients associated with mammalian wounds is within the range of fields found to influence development (Jaffe & Vanable 1984). It is suggested that these wound currents could be responsible for stimulating wound cover by epithelial cells (Foulds & Barker 1983, Jaffe & Vanable 1984). The stimulation of skin lesions by externally applied electrical currents is gaining clinical acceptance and is in itself a considerable field of study (Vanable 1989, Watson 1996).
Illingworth & Barker (1980) have demonstrated local currents associated with amputated fingertips in children. The current density was on average 22μA cm−2 and reached its peak approximately 1 week after the injury. The fingertips regenerated if left unsutured and the battery function of the skin was found to be comparable to that involved in amphibian limb regeneration. The skin battery current driving capacity is in the order of 1 μA/mm of wound length (Borgens 1982, Jaffe & Vanable 1984, Vanable 1989).
An extensive literature has demonstrated the relationship between skin injury and changes in bioelectric activity (e.g. Vanable 1989, Watson 1995) and it would take at least a whole chapter to provide a minimal review. There is a strong relationship between this normal level of skin electrical activity and the levels of current/potential in wounds that are not healing. Just as was the case with the delayed or non-unions in bone, the equivalent in the skin is the chronic, non-healing or slowly healing ulcer or pressure sore.
In the same way that the repair process can be stimulated in bone by various means, including electrotherapy in the form of ultrasound, laser and electrical stimulation, it is also the case with chronic venous ulcers and equivalent skin lesions. Chapter 19 covers the use of electrical stimulation as a means to enhance the local bioelectric activity in open wounds, and Chapters 10, 11 and 12, concerned with pulsed shortwave, laser and ultrasound, make mention of their role in this context.
The use of electrical stimulation in wound management is not common practice in the UK, although in some countries it has been adopted as a matter of routine and thousands of patients benefit from this intervention (Kloth 2005).
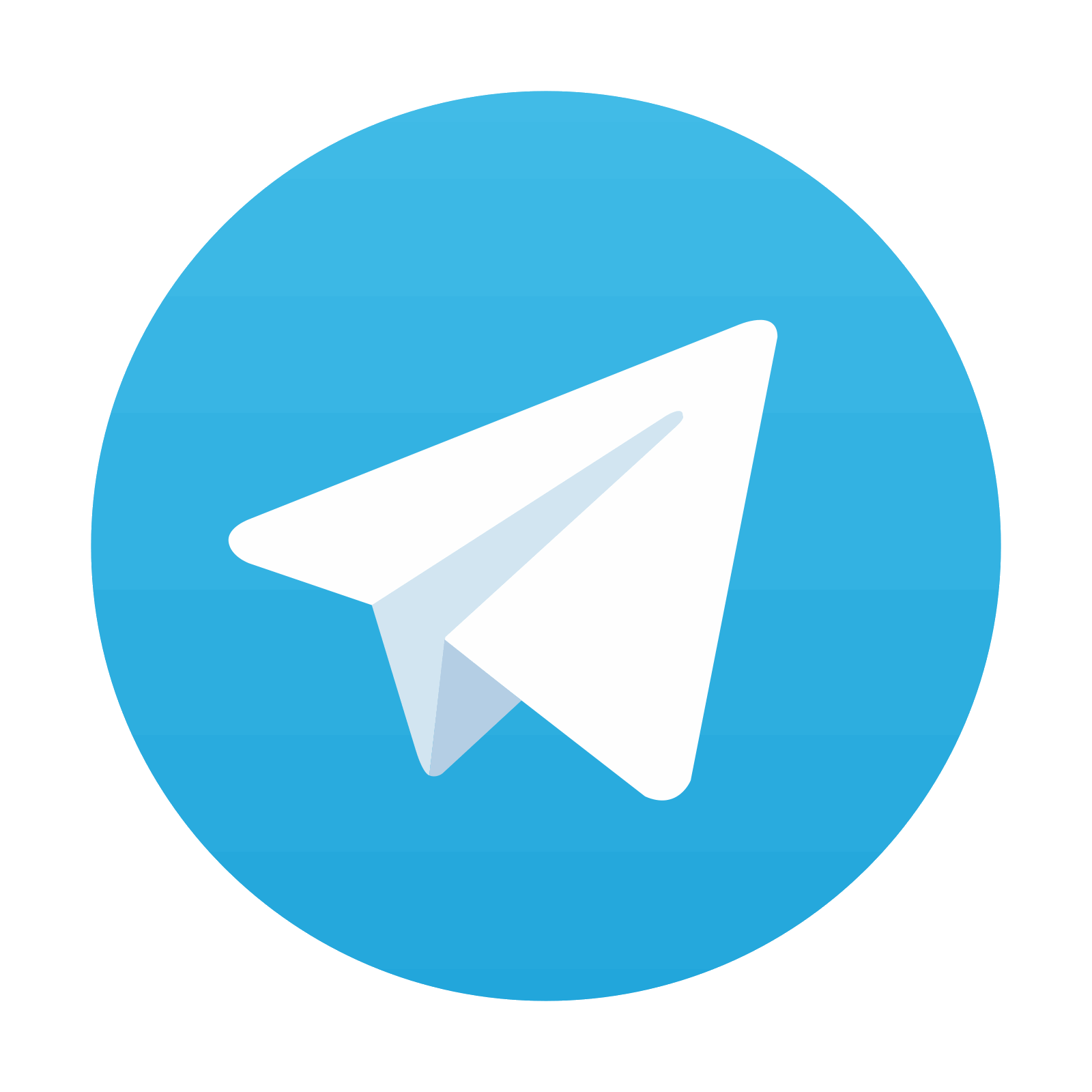
Stay updated, free articles. Join our Telegram channel
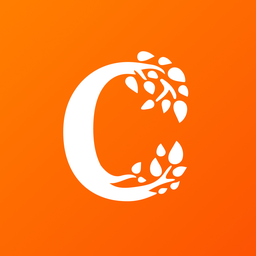
Full access? Get Clinical Tree
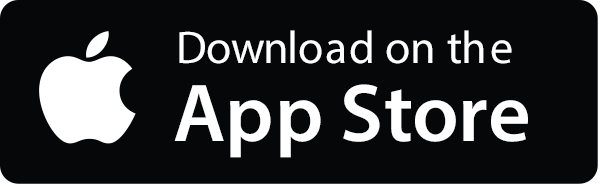
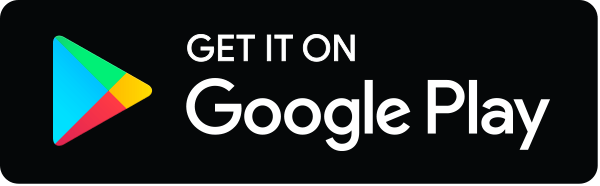