The standard of care for newly diagnosed malignant glioblastoma entails postoperative radiotherapy and adjuvant chemotherapy with temozolomide. There has been an increase in the incidence of enhancing and progressive lesions seen on magnetic resonance imaging (MRI) following treatment. Conventional MRI with gadolinium contrast is unable to distinguish between the effects of treatment and actual tumor recurrence. New modalities have provided additional information for distinguishing treatment effects from tumor progression but are not 100% sensitive or specific in diagnosing progression. Novel radiographic or nonradiographic biomarkers with sensitivity and specificity verified in large randomized clinical trials are needed to detect progression.
The median survival from the time patients are diagnosed with glioblastoma, the most common malignant primary tumor, was improved to 15 months with the introduction of the Stupp protocol in 2005 and has been improved to 23 months in small retrospective series with the aggressive use of more advanced therapeutic modalities at the time of recurrence, like antiangiogenic therapy with vascular endothelial growth factor (VEGF) neutralizing antibody bevacizumab.
The Stupp protocol was established in a phase II, randomized clinical trial by Stupp and colleagues and involves administering a total of 60 Gy of radiation during 30 sessions spanning a 6-week period while treating simultaneously with 75 mg/m 2 of temozolomide on a daily basis. Adjuvant temozolomide chemotherapy is then administered in six 28-day cycles with a dose of 150 mg/m 2 for the initial cycle followed by 200 mg/m 2 for the remaining 5 cycles. Despite the improved survival rate established by the Stupp protocol, most patients continue to experience tumor recurrence, which necessitates close follow-up with magnetic resonance imaging (MRI). Although many have reported that there is no difference in the percentage of recurrence in distal locations after the Stupp protocol treatment, others have suggested an increased proportion of distal recurrence in patients being treated with temozolomide as compared with radiotherapy alone.
Antiangiogenic therapy became a mainstay in the treatment of recurrent glioblastoma since a pair of phase II clinical trials showed efficacy of bevacizumab and irinotecan in treating recurrent glioblastoma, leading to the 2009 accelerated Food and Drug Administration approval of bevacizumab for recurrent glioblastoma, with a pair of large, randomized, phase III clinical trials underway evaluating the efficacy of bevacizumab in treating newly diagnosed glioblastoma.
Clinicians have long noted common transient impacts of radiation on MRI contrast enhancement. When occurring in the first 3 months after radiation, this effect imitates early tumor progression and has earned the term pseudoprogression. Another effect witnessed after therapy is lesion enhancement in patients undergoing cranial radiation, which takes place in 3% to 24% of patients 3 to 12 months after treatment and is referred to as radiation necrosis. Additionally, the identification of nonenhancing fluid-attenuated inversion recovery (FLAIR) bright infiltrative growth representing glioblastoma recurrence after antiangiogenic therapy has cofounded the ability of clinicians to recognize glioblastoma recurrence using conventional MRI and the Macdonald criteria, which are based on gadolinium enhancement. The goal of this article is to review the most recent advancements made in radiology and cancer biology that can be used to improve the ability of physicians to distinguish true recurrence from pseudoprogression or radiation-induced necrosis in patients with glioblastoma receiving radiation, temozolomide, or antiangiogenic therapy.
Pseudoprogression: early transient enhancing lesions
Pseudoprogression is defined as enhancing changes seen on MRI within the first 3 months after treatment of glioblastoma with fractionated radiotherapy. Pseudoprogression represents treatment effects rather than treatment failure, which, with time, either successively recovers or stabilizes without being linked to subordinate outcomes. Since postoperative radiotherapy and concomitant temozolomide were established as the standard treatment of glioblastoma, there has been an increase in pseudoprogression from 10% in patients with glioblastoma treated with radiotherapy alone up to nearly 30% in patients treated with radiotherapy and concomitant chemotherapy.
Pseudoprogression is a transient process that results from increased permeability and leakage of gadolinium through both the dysfunctional blood-brain barrier in the irradiated region as well as the capillary bed of the tumor. Pseudoprogression reflects response to treatment rather than treatment failure and with time it improves or stabilizes and has not shown to have any correlation with poorer outcomes and in some studies has been shown to be associated with improved outcomes. Based on postoperative MRI studies, pseudoprogression occurs within the first 2 months following treatment as opposed to radiation necrosis, which can take an average of 3 to 12 months before it is witnessed on imaging. The increase in incidence of pseudoprogression since the advent of simultaneous administration of chemotherapy and radiotherapy has been suggested to be a marker for improved response to treatment, a hypothesis supported by a study in which patients with glioblastoma with pseudoprogression had greater overall survival than those without pseudoprogression.
Three factors have been proposed in the literature to increase the incidence of pseudoprogression in patients with glioblastoma: methylated O6—methyl guanine-DNA methyl transferase (MGMT) status, higher doses of radiation, and concomitant administration of chemotherapy and radiation.
Temozolomide is an alkylating cytostatic prodrug that undergoes rapid conversion and is hydrolyzed to its active metabolite, 3-methyl-(triazen-1-yl)imidazole-4-carboximide (MTIC). MTIC functions to methylate DNA at several positions, the most important of which is the O6 position of guanine, which leads to cell death. MGMT is an enzyme that removes this methylation to prevent the lethal DNA damage the cell would otherwise sustain. Cells that have epigenetic silencing of the MGMT gene, achieved through promoter methylation, are unable to produce the enzyme and are, therefore, more sensitive and responsive to the temozolomide-induced cell death. One study was able to demonstrate that 66% of their patients with pseudoprogression had methylated MGMT promoters, whereas 89% of patients who had true progression while on temozolomide showed unmethylated MGMT promoters. This study was able to establish pseudoprogression to be more common with a ratio of 2:1 in patients whose tumors exhibited methylated MGMT promoters, whereas disease progression is more likely with a ratio of 3:1 in patients whose tumors exhibited unmethylated MGMT promoters.
Since MGMT promoter methylation status has been proven to serve as a marker for pseudoprogression, there has been a wide search for a highly sensitive and specific screening assay. Currently, methylation-specific polymerase chain reaction (MSP) is the standard method used to test for MGMT promoter methylation status. The biggest drawback for this method is its inability to provide quantitative results, which leads to some inaccuracy predicting prognosis, and the likelihood of pseudoporgression. Methylation-specific multiplex ligation probe amplification (MS-MLPA) has been recently introduced as a semiquantitative method for testing methylation status of multiples genes in a simultaneous fashion while also proving to be a consistent method for determining the methylation status of MGMT promoter in tumor tissue. One recent study found that the combination of MSP and MS-MLPA can further augment the diagnostic accuracy of pseudoprogression to 93%, which could have a significant impact on clinical decisions.
Radiation necrosis: delayed enhancing lesions
Since the randomized clinical trials in the 1970s demonstrating an improvement in overall survival, postoperative whole-brain radiotherapy at a daily dose of 2 Gy per fraction given over a 6-week period for a total dose of 60 Gy has become part of the standard treatment of glioblastoma. Patients treated with radiation therapy in this manner often exhibit enhancement in radiation within 3 to 12 months of completing radiation. This phenomenon is called radiation necrosis and is now a common manifestation of radiotherapy seen in 3% to 24% of cases, with an even higher incidence noted in patients receiving temozolomide treatment concurrent to radiotherapy. The enhancement seen on conventional MRI may be secondary to the radiation-induced damage of the blood-brain barrier, which causes gadolinium leakage into the interstitium and produces a ring-enhancing lesion that can be easily misread as tumor recurrence.
Radiation necrosis can be witnessed as early as 3 months and its presence has been reported as late as 5 years after the original treatment, although most cases typically occur within the first 2 years after radiation. One study reports the incidence of radiation necrosis in patients with glioblastoma treated at 60 Gy with conventional fractionated external beam radiotherapy to be just at 1%, but as mentioned previously, there has been an increase in its incidence since the introduction of adjuvant chemotherapeutic agents, such as temozolomide. Radiation-induced necrosis most commonly occurs at the location that has received the maximum dose of radiation, which usually falls in the purlieu of the tumor site and close to the margins of the surgical cavity of the resected tumor. The periventricular white matter is known to be a common location for the occurrence of radionecrosis and is highly vulnerable to ischemic effects of postradiation vasculopathy, a phenomenon which may be explained by the poor blood supply this location receives from long medullary arteries lacking collateral vessels.
The clinical presentation of radiation necrosis often mimics that of tumor recurrence. Patients can present with a broad set of symptoms, such as headache, seizures, behavioral changes, focal neurologic deficits, wheras some may be asymptomatic. It is imperative to distinguish between the recurrence and radiation necrosis because the approach to treatment is fairly specific to each and can lead to unnecessary morbidity and even mortality if the incorrect treatment modality is pursued. A common finding using conventional MRI of radiation necrosis may be a mass of enhancing lesions seen on T1-weighted imaging with gadolinium contrast administration, but there is no imaging correlation that can differentiate radiation necrosis from true progression or pseudoprogression, especially on the initial postradiotherapy temozolomide scan. The main shortcoming of conventional MRI, which limits its ability to distinguish viable tumor mass from radiation necrosis, is that it only recognizes disruptions made to the blood-brain barrier and edema. In a previous study looking for specific markers of radiation necrosis on conventional MRI, individual signs were not found to be valuable markers of tumor recurrence, whereas the combination of 2 signs in addition to the involvement of the corpus callosum and numerous enhancing lesions was a statistically significant marker for recurrence. A more recent study that used a larger sample size was able to recognize subependymal enhancement as an individual marker for the early progression of tumor on conventional MRI. Even though this marker was statistically significant, the investigators from the study recognize that radiation necrosis at higher doses may lead to radiographic findings that can mimic subependymal enhancement, hence, its less than perfect ability to serve as a definitive marker for recurrence.
Several clinical factors have been associated with an increase in the risk of radiation necrosis in patients with glioma, which include the following: older age, high fraction doses (<2.5 Gy/d), hyperfractionation, lower conformity index, shorter overall treatment time, stereotactic radiosurgery, interstitial brachytherapy, reirradiation, and radiation combined with adjuvant chemotherapy. Radiation necrosis is directly related to the volume of irradiated brain in addition to the dose of radiation that is delivered, with a precipitous increase with doses exceeding 65 Gy in fractions of 1.8 to 2.0 Gy. One study found the administration of chemotherapy alongside radiation increases the risk of cerebral necrosis fourfold. Furthermore, the brain parenchyma surrounding a glioma has been shown to be particularly sensitive to chemotherapy and radiation. Such risks must be taken into account when making a distinction between treatment effects versus tumor recurrence.
Tumor cells have been shown to be interspersed in many histologic specimens of radiated necrotic tissue, with one study showing that 55% of pathologically examined cases of radiation necrosis demonstrated viable tumor coexistence within areas of necrosis. To determine whether chemotherapy should be continued or switched, the percentage of viable tumor cells versus necrotic tissue in the specimen is taken into account, with the threshold for switching chemotherapy being met typically if the specimen contains greater than 50% viable tumor cells.
Pathophysiology of Radiation Necrosis
The histopathologic features observed in radiation necrosis are demonstrated in Fig. 1 . Vascular endothelial cells and oligodendrocytes have been shown to serve as the direct targets of radiation in the central nervous system. These 2 targets are directly injured and independently lead to necrosis (see Fig. 1 A) and demyelination both within the normal tissue and the tumor. The development of acute and subacute radiation injury is secondary to the clonogenic death of endothelial cells, and vascular lesions also play a key role in late radiation injury. The direct consequence of endothelial cell death from radiation is thought to induce the breakdown of the blood-brain barrier leading to ischemia, vasogenic edema, and hypoxia. The hypoxic tissue then upregulates VEGF, which plays a major role in increasing vascular permeability and is shortly followed by tissue necrosis and demyelination (see Fig. 1 B). Unfortunately, radiotherapy has also been shown to stimulate glioma cells to secrete an increased amount of VEGF, causing a decrease in apoptosis in both endothelial cells and tumor cells while increasing angiogenesis in the tumor environment (see Fig. 1 C), which is a mechanism that may account for the radiation-resistance that develops in gliomas. The increase in VEGF production can be counteracted by the addition of bevacizumab, an antibody to VEGF that is now used frequently in cases of glioblastoma recurrence. Therefore, bevacizumab can function to decrease enhancement and edema while substantially improving the radiographic appearance of radiation necrosis, which mimics tumor progression.
The mechanism by which radiation induces endothelial cell apoptosis is largely caused by damage to the cell membrane, although DNA damage still plays a role. The incited damage to the cellular membrane activates acid sphingomyelinase, which is an enzyme involved in sphingolipid metabolism and produces ceramide, a bioactive lipid well known for its physiologic role in apoptosis. Radiation-induced DNA damage activates ceramide synthase, which serves as an additional source of ceramide, which causes additional endothelial cell apoptosis through the P53-dependent stimulation of the mitochondrial and death-receptor pathways.
The current understanding of the pathophysiology of radiation-induced necrosis may be further used for treatment and molecular prevention of the radiologic and clinical effects of radiation necrosis. In one study, protein kinase C (PKC) was shown to prevent radiation damage because the downregulation of acid sphingomyelinase is a PKC-dependent mechanism, whereas PKC also functions to inhibit ceramide-dependent apoptosis. In a study conducted by Fuks and colleagues, basic fibroblast growth factor was used to stimulate PKC activity, allowing for the inhibition of radiation-induced endothelial cells in both in vivo and in vitro experiments.
Radiation necrosis: delayed enhancing lesions
Since the randomized clinical trials in the 1970s demonstrating an improvement in overall survival, postoperative whole-brain radiotherapy at a daily dose of 2 Gy per fraction given over a 6-week period for a total dose of 60 Gy has become part of the standard treatment of glioblastoma. Patients treated with radiation therapy in this manner often exhibit enhancement in radiation within 3 to 12 months of completing radiation. This phenomenon is called radiation necrosis and is now a common manifestation of radiotherapy seen in 3% to 24% of cases, with an even higher incidence noted in patients receiving temozolomide treatment concurrent to radiotherapy. The enhancement seen on conventional MRI may be secondary to the radiation-induced damage of the blood-brain barrier, which causes gadolinium leakage into the interstitium and produces a ring-enhancing lesion that can be easily misread as tumor recurrence.
Radiation necrosis can be witnessed as early as 3 months and its presence has been reported as late as 5 years after the original treatment, although most cases typically occur within the first 2 years after radiation. One study reports the incidence of radiation necrosis in patients with glioblastoma treated at 60 Gy with conventional fractionated external beam radiotherapy to be just at 1%, but as mentioned previously, there has been an increase in its incidence since the introduction of adjuvant chemotherapeutic agents, such as temozolomide. Radiation-induced necrosis most commonly occurs at the location that has received the maximum dose of radiation, which usually falls in the purlieu of the tumor site and close to the margins of the surgical cavity of the resected tumor. The periventricular white matter is known to be a common location for the occurrence of radionecrosis and is highly vulnerable to ischemic effects of postradiation vasculopathy, a phenomenon which may be explained by the poor blood supply this location receives from long medullary arteries lacking collateral vessels.
The clinical presentation of radiation necrosis often mimics that of tumor recurrence. Patients can present with a broad set of symptoms, such as headache, seizures, behavioral changes, focal neurologic deficits, wheras some may be asymptomatic. It is imperative to distinguish between the recurrence and radiation necrosis because the approach to treatment is fairly specific to each and can lead to unnecessary morbidity and even mortality if the incorrect treatment modality is pursued. A common finding using conventional MRI of radiation necrosis may be a mass of enhancing lesions seen on T1-weighted imaging with gadolinium contrast administration, but there is no imaging correlation that can differentiate radiation necrosis from true progression or pseudoprogression, especially on the initial postradiotherapy temozolomide scan. The main shortcoming of conventional MRI, which limits its ability to distinguish viable tumor mass from radiation necrosis, is that it only recognizes disruptions made to the blood-brain barrier and edema. In a previous study looking for specific markers of radiation necrosis on conventional MRI, individual signs were not found to be valuable markers of tumor recurrence, whereas the combination of 2 signs in addition to the involvement of the corpus callosum and numerous enhancing lesions was a statistically significant marker for recurrence. A more recent study that used a larger sample size was able to recognize subependymal enhancement as an individual marker for the early progression of tumor on conventional MRI. Even though this marker was statistically significant, the investigators from the study recognize that radiation necrosis at higher doses may lead to radiographic findings that can mimic subependymal enhancement, hence, its less than perfect ability to serve as a definitive marker for recurrence.
Several clinical factors have been associated with an increase in the risk of radiation necrosis in patients with glioma, which include the following: older age, high fraction doses (<2.5 Gy/d), hyperfractionation, lower conformity index, shorter overall treatment time, stereotactic radiosurgery, interstitial brachytherapy, reirradiation, and radiation combined with adjuvant chemotherapy. Radiation necrosis is directly related to the volume of irradiated brain in addition to the dose of radiation that is delivered, with a precipitous increase with doses exceeding 65 Gy in fractions of 1.8 to 2.0 Gy. One study found the administration of chemotherapy alongside radiation increases the risk of cerebral necrosis fourfold. Furthermore, the brain parenchyma surrounding a glioma has been shown to be particularly sensitive to chemotherapy and radiation. Such risks must be taken into account when making a distinction between treatment effects versus tumor recurrence.
Tumor cells have been shown to be interspersed in many histologic specimens of radiated necrotic tissue, with one study showing that 55% of pathologically examined cases of radiation necrosis demonstrated viable tumor coexistence within areas of necrosis. To determine whether chemotherapy should be continued or switched, the percentage of viable tumor cells versus necrotic tissue in the specimen is taken into account, with the threshold for switching chemotherapy being met typically if the specimen contains greater than 50% viable tumor cells.
Pathophysiology of Radiation Necrosis
The histopathologic features observed in radiation necrosis are demonstrated in Fig. 1 . Vascular endothelial cells and oligodendrocytes have been shown to serve as the direct targets of radiation in the central nervous system. These 2 targets are directly injured and independently lead to necrosis (see Fig. 1 A) and demyelination both within the normal tissue and the tumor. The development of acute and subacute radiation injury is secondary to the clonogenic death of endothelial cells, and vascular lesions also play a key role in late radiation injury. The direct consequence of endothelial cell death from radiation is thought to induce the breakdown of the blood-brain barrier leading to ischemia, vasogenic edema, and hypoxia. The hypoxic tissue then upregulates VEGF, which plays a major role in increasing vascular permeability and is shortly followed by tissue necrosis and demyelination (see Fig. 1 B). Unfortunately, radiotherapy has also been shown to stimulate glioma cells to secrete an increased amount of VEGF, causing a decrease in apoptosis in both endothelial cells and tumor cells while increasing angiogenesis in the tumor environment (see Fig. 1 C), which is a mechanism that may account for the radiation-resistance that develops in gliomas. The increase in VEGF production can be counteracted by the addition of bevacizumab, an antibody to VEGF that is now used frequently in cases of glioblastoma recurrence. Therefore, bevacizumab can function to decrease enhancement and edema while substantially improving the radiographic appearance of radiation necrosis, which mimics tumor progression.
The mechanism by which radiation induces endothelial cell apoptosis is largely caused by damage to the cell membrane, although DNA damage still plays a role. The incited damage to the cellular membrane activates acid sphingomyelinase, which is an enzyme involved in sphingolipid metabolism and produces ceramide, a bioactive lipid well known for its physiologic role in apoptosis. Radiation-induced DNA damage activates ceramide synthase, which serves as an additional source of ceramide, which causes additional endothelial cell apoptosis through the P53-dependent stimulation of the mitochondrial and death-receptor pathways.
The current understanding of the pathophysiology of radiation-induced necrosis may be further used for treatment and molecular prevention of the radiologic and clinical effects of radiation necrosis. In one study, protein kinase C (PKC) was shown to prevent radiation damage because the downregulation of acid sphingomyelinase is a PKC-dependent mechanism, whereas PKC also functions to inhibit ceramide-dependent apoptosis. In a study conducted by Fuks and colleagues, basic fibroblast growth factor was used to stimulate PKC activity, allowing for the inhibition of radiation-induced endothelial cells in both in vivo and in vitro experiments.
Antiangiogenic therapy for glioblastoma: radiologic and nonradiologic biomarkers of efficacy
Bevacizumab, a monoclonal anti-VEGF antibody, has been studied in phase II trials of patients with recurrent glioblastoma and demonstrated a 23-week medial progression-free survival when administered in combination with irinotecan. A study of patients with glioblastoma treated with bevacizumab revealed decreased tumor hypoxia and increased tumoral VEGF expression, which were directly associated with radiographic response and prolonged survival following treatment.
VEGF levels have been evaluated in the plasma and tumor fluid samples from patients with glioblastoma, which showed a relative elevation, whereas levels within the tumor cavity were elevated even higher in patients experiencing recurrence of the disease as compared with those with stable disease after resection. Furthermore, a direct correlation has been established between VEGF overexpression and poor prognosis based on glioblastoma tumor histology. Antiangiogenic therapy has been proposed to normalize the vasculature within the tumor, allowing for thinning of the superfluous perivascular and endothelial cells and lessening the tortuosity of blood vessels while reducing the interstitial pressure. This decrease in pressure is suggested to enhance the transport of chemotherapy to the tumor cells while improving oxygenation. Using bevacizumab as the agent for VEGF blockade, another study demonstrated a reduction of the microvascular density while improving the function of intratumoral vessels, which enables the penetration of chemotherapy.
Unfortunately, anti-VEGF treatment in animal models has shown to increase the infiltrative growth capability of the tumor, which allows for bright recurrent lesions on FLAIR MRI sequencing but is nonenhancing because of their decreased vascular permeability.
Impact of Antiangiogenic Therapy on Imaging
Typically, the abnormal vasculature along with the highly permeable blood-brain barrier of glioblastoma permits leakage of contrast material from the tumor capillaries, which leads to an amplified enhancement of T1-weighted imaging ( Fig. 2 ). Antiangiogenic agents, such as bevacizumab, decrease the leak of contrast agent into the interstitium, which markedly decreases contrast enhancement. Therefore, a decrease in contrast enhancement of the tumor does not reflect a true cytostatic or cytotoxic tumor, and the dependence on contrast enhancement alone can cause an overestimation of the response to treatment, which has earned the term pseudoresponse, representing in effect the converse of the pseudoprogression described earlier. The occurrence of radiation necrosis further takes away from determining the true response of glioblastoma to antiangiogenic therapy because it is speculated that at least part of the radiologic response witnessed following bevacizumab therapy is caused by the palliation of radiation necrosis as opposed to a true tumor response. These barriers have necessitated new imaging modalities that can better assess tumor response in patients receiving antiangiogenic therapy. To make this task a reality, such techniques will need the ability to distinguish true tumor progression from pseudoprogression while unraveling nonenhancing tumor from gliosis. Some of these novel techniques will be introduced and discussed later.
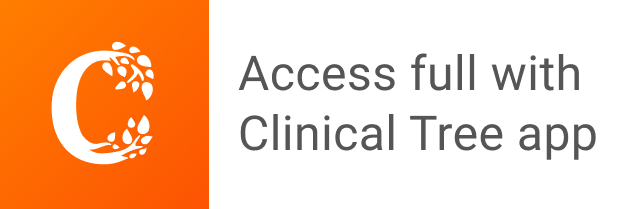